Recycling valuable materials from the spent lithium ion batteries to catalysts: methods, applications, and characterization
Abstract
As the prevailing technology for energy storage, the extensive adoption of lithium-ion batteries (LIBs) inevitably results in the accumulation of numerous spent batteries at the end of their lifecycle. From the standpoints of environmental protection and resource sustainability, recycling emerges as an essential strategy to effectively manage end-of-life LIBs and reclaim valuable elements within them. Hydrometallurgy, closely intertwined with catalysis, stands as a relatively mature strategy for achieving high-value utilization of spent LIBs. In this review, our emphasis is placed on the interconnected themes of catalysis within the realm of hydrometallurgical recycling. Specifically, we delve into the crucial role that catalysis plays in both the recycling process of LIBs and the sustainable utilization of their extracted materials in various catalytic applications. This focused exploration aims to contribute insights into the intricate relationship between catalysis and the broader context of LIB recycling, shedding light on its pivotal role in achieving both environmental sustainability and functional material repurposing. Moreover, we highlight advanced characterization techniques, represented by surface-sensitive enhanced Raman spectroscopy, to fundamentally understand the reaction mechanism of catalysts, which, in turn, would inform more rational catalyst designs.
Keywords
INTRODUCTION
Over the past two centuries, fossil energy has been instrumental in propelling the advancement of human civilization while providing essential support for economic and social development. However, as the finite and non-renewable nature of fossil energy becomes increasingly apparent, humanity has proactively pivoted towards exploring and exploiting renewable energy sources[1]. Concurrently, there has been notable progress in energy storage technologies, encompassing mechanical, electrochemical, and thermal storage methods, among others[2]. Electrochemical energy storage, exemplified by lithium-ion batteries (LIBs), stands out as a prominent focal point in the ongoing global energy transition[3]. LIBs have achieved successful industrialization and found widespread applications in diverse fields such as intelligent devices, electric vehicles, aerospace, and more. This popularity stems from their numerous advantages, including high voltage, substantial specific energy, commendable safety performance, rapid charge/discharge capabilities, and a broad operating temperature range.
The fundamental structure of a LIB is characterized by essential components involving the cathode, anode, current collector, separator, and electrolyte, each composed of a diverse array of chemical elements. However, in practical applications, these foundational elements are intricately integrated to create complex battery structures, encompassing various designs such as coin-type, cylindrical-type, prismatic-type, and more. This amalgamation ensures the stable charge/discharge performance of the battery across diverse usage scenarios and applications. Regardless of the configurations, LIBs have a limited lifespan from a practical point of view, necessitating disposal when their capacity diminishes to less than 80%[4]. In the upcoming years, a considerable number of actively used LIBs will face renewal, potentially triggering a crisis of battery resource waste. Importantly, the inadequate recycling of specific materials within LIBs poses a significant risk of water and soil pollution when these batteries are exposed to the natural environment[5]. In fact, LIBs house valuable elements such as Li, Ni, Co, and Mn, which are integral components of effective catalysts[6,7]. Ensuring the proper recycling and utilization of these materials becomes not only a means of mitigating environmental hazards but also a commitment to the principles of resource sustainability and economic benefit. Effectively managing the impending surge of spent LIBs is crucial for charting a course toward a more sustainable and environmentally conscious future[8,9].
The recycling stream of LIBs is typically segmented into three stages: upstream, midstream, and downstream. Batteries scrapped from electronic devices in the upstream stage undergo recycling and processing in the midstream, ultimately reintegrating into use in the downstream. A portion of spent batteries retaining high specific energy and relatively intact structures can be strategically repurposed through gradient utilization[10]. These batteries find applications in areas such as low-speed electric vehicles, large-scale energy storage equipment, communication base stations, and other sectors where stringent battery performance requirements are not critical. Conversely, batteries exhibiting a significant decline in specific energy and extensive structural damage necessitate recycling for their constituent materials. Crucially, the midstream and downstream stages are intricately linked to the “catalysis”. The primary methods employed in midstream recycling encompass pyrometallurgy[11-13], hydrometallurgy[14-17], and direct recycling[18-23]. Direct recycling emerges as a non-destructive method that directly collects and rejuvenates active electrode materials for LIBs. This approach encompasses four distinct recovery techniques: solid-state, electrochemical, solution-based, and eutectic salt relithiation[24]. Among these approaches, eutectic salt recrystallization stands out for its effective recovery of spent battery materials, leveraging the self-saturation property. This makes it the more popular direct recovery method currently. By blending various salts to lower the eutectic point, this technique not only replenishes the anode with lithium but also rejuvenates the cathode. Despite its high efficiency and energy-saving attributes, direct recycling is currently confined to laboratory stages and has not yet been industrialized. The pyrometallurgy and hydrometallurgy constitute comparatively straightforward procedures with high recovery efficiency, making them prevalent in the field of LIB recycling. Pyrometallurgy encompasses three main steps: roasting, smelting, and refining, involving high-temperature processing, which can result in significant energy consumption and potential air pollution[25]. Nevertheless, it continues to play a significant role in today’s recycling of used batteries due to its straightforward nature. Furthermore, continuous improvements have been made in pyrometallurgical processes, with researchers prioritizing energy consumption reduction. Techniques such as microwave-assisted carbothermal reduction or salt-assisted reduction roasting in the roasting stage have been developed, enabling the reduction of high-valent metals to low-valent metals at lower reaction temperatures. This reduction not only facilitates easier leaching of metals but also enhances environmental friendliness. In contrast, hydrometallurgical process, employing low-temperature leaching and subsequent separation and purification, effectively extracts valuable metals from spent LIBs. This method has matured over time, and the integration of catalytic methods during the leaching process ensures a high leaching rate while maintaining an environmentally friendly and sustainable approach. On the other hand, the metal-rich leachate obtained through recovery can undergo further regeneration through diverse techniques to yield corresponding metal salts or metal oxides. These materials can act as precursors for synthesizing high-performance catalysts or be directly utilized as catalysts themselves. Their primary applications range from environmental pollution degradation, remediation of harmful substances in water bodies, electrocatalytic oxidation, etc. As a result, the hydrometallurgy, closely intertwined with catalysis, is currently a promising strategy for achieving high-value utilization of spent LIBs. Therefore, an in-depth review of existing catalytic methods within hydrometallurgical approaches for recycling spent LIBs, along with the subsequent application of recycled products in catalytic processes, is essential to establish a common knowledge framework in this research area.
In this review, we focus on the interconnected themes of catalysis within the realm of LIB recycling, especially the pivotal role catalysis plays in both the recycling processes of LIBs and the sustainable utilization of their extracted materials across various catalytic applications. First, several catalytic strategies for extracting metals from spent LIBs are presented. Subsequently, the recyclable components derived from cathodes and/or anodes, along with their applications in diverse catalytic fields, are systematically summarized. Moreover, advanced spectroscopic characterization techniques, exemplified by X-ray-based techniques and Raman spectroscopy, are underscored for their capability to enhance the fundamental understanding of catalyst structure and catalytic mechanisms. This focused exploration aims to provide valuable insights into the intricate relationship between catalysis and the broader context of LIB recycling, thereby illuminating its crucial role in achieving both environmental sustainability and the effective repurposing of functional materials. This delimitation distinguishes this review from existing articles that have broader scopes and are more comprehensive. For example, the readers can refer to ref.[26-30] for an overview of the current process for LIB recycling.
CATALYTIC STRATEGIES APPLIED IN RECYCLING OF LI-ION BATTERIES
For the recycling of spent LIBs, hydrometallurgy, primarily relying on leaching, emerges as a well-established technique. The leaching process involves the application of inorganic/organic acids and bases to dissolve metal oxides, followed by obtaining corresponding metal salts through stepwise precipitation. However, this process is often constrained by experimental conditions, such as pH, temperature, and leaching time. By catalyzing this reaction through external means, the energy consumption of the process can be minimized, facilitating a substantial improvement in leaching kinetics, metal leaching rates, and even selective leaching of metals. This catalytic intervention proves particularly effective under mild acidic and alkaline conditions, holding significant implications for promoting environmental sustainability. In this section, we highlight three recently developed catalysis-assisted strategies for enhancing the LIB recycling efficiency.
Photocatalysis-assisted recycling
Photocatalysis is a chemical reaction facilitated by light[31,32]. This photochemical process involves molecules absorbing specific wavelengths of electromagnetic radiation, inducing molecular excited states. Subsequently, a chemical reaction occurs, forming new substances or intermediate chemical products that can initiate thermal reactions. The activation energy for photochemical reactions is derived from the energy of photons. Ultraviolet (UV) light, characterized by its potent photon energy, serves as an effective photocatalyst, enhancing the overall efficiency of the reaction.
Lv et al. utilized UV-assisted leaching for recycling cathodes in spent LIBs, employing H2O2 widely used in hydrometallurgy as a reductant [Figure 1A][33]. Under UV irradiation, the excitation of unstable O–O and
Figure 1. Three catalytic methods applied in hydrometallurgy recovery processes. (A) Schematic diagram of photocatalysis-assisted recycling. Quoted with permission from Lv et al.[33]; (B) Illustration outlining the CEC-leaching strategy. Quoted with permission from Li et al.[37]; (C) Schematic diagram of alkali metal catalytic carbothermal reduction. Quoted with permission from Zhang et al.[38]. CEC: Contact-electro-catalysis. UV: ultraviolet; LCO: lithium cobalt oxide.
Ultrasound-assisted recycling
Ultrasound-assisted leaching is another popular technique in hydrometallurgy for recycling spent LIBs. Almost all ultrasonication techniques performed in liquids are associated with cavitation[34]. Ultrasonic cavitation occurs when the ultrasonic energy is sufficiently high, causing tiny bubbles (cavitation nuclei) in the liquid to vibrate, grow, and continuously gather sound field energy. When the energy reaches a certain threshold, the cavitation bubbles dramatically collapse and close. Ultrasonic cavitation serves to accelerate chemical reactions and can also control the reaction process, enhancing yield while reducing side reactions. In certain cases, reactions that are difficult to occur under normal circumstances can proceed smoothly under ultrasonic irradiation.
Jiang et al. employed an ultrasound-assisted leaching method to recover Li and Co from spent LIBs, using H2SO4-H2O2 as a leaching agent[35]. Compared to conventional leaching methods, this approach achieved a high leaching rate, with improved Li and Co leaching efficiencies reaching up to 98.62% and 94.63%, respectively, under an ultrasonic power of 360 W with leaching time of 30 min. Similarly, Li et al. applied an ultrasound-assisted strategy to leaching Li and Co using citric acid [Figure 1B][36]. Due to the unique ultrasonic cavitation effect, the optimized leaching efficiencies for the two elements reached as high as 100% and 96%, respectively, within 5 h using a lower ultrasonic power of 90 W. Recently, Li et al. proposed a mechano-catalytic method, termed contact-electro-catalysis (CEC), for the spent LIB recovery, which utilizes free radicals generated by contact electrification to promote metal leaching in the presence of an ultrasound-assisted scenario[37]. For spent LiCoO2 batteries, the leaching efficiencies of 100% for Li and 92.19% for Co were achieved within 6 h at 90 °C. In contrast, for ternary Li batteries, the leaching efficiencies within 6 h at 70 °C were 94.56% for Li, 96.62% for Ni, 96.54% for Mn, and 98.39% for Co, respectively. The CEC-leaching strategy offers a green, efficient, and economical approach to recovering LIBs, which is expected to meet the growing demand for LIB recycling.
Metal catalyst-assisted recycling
Adding metal catalysts to the leaching system, capitalizing on their excellent catalytic activity, can significantly enhance the leaching rate and efficiency. Zhang et al. employed alkali metals as catalysts, such as NaOH, significantly reducing the temperature needed for carbothermic reduction of spent
APPLICATION OF EXTRACTED MATERIALS FROM SPENT LIBS IN CATALYTIC FIELDS
By recovering metals, such as Li, Ni, Co, Mn, Cu, and Fe, from cathodes and anodes in spent LIBs, various highly efficient catalysts can be prepared for applications including the degradation of toxic organics in the environment, electrocatalysis, and air batteries, etc. The performance of these obtained catalysts is comparable to or even surpasses that of commercial catalysts, showcasing significant potential and economic benefits. To facilitate discussion, this section delves into the potential extraction of materials and their applications in different catalytic fields, categorized by electrodes.
Cathode
The valuable metals Ni, Co, Mn, and Fe possessing commendable catalytic properties, obtained by recycling around the common cathodes in spent LIBs, can be converted into catalysts. Moreover, the catalyst can undergo modification through multiple metal doping, resulting in an improved catalyst that exhibits excellent catalytic activity [Table 1].
Summary of catalysts made from spent LIBs
Electrode | Materials | Catalyst | Application | Ref. |
Cathode | LCO | 94% LiCoO2 and 6% Co3O4 | Decomposition of VOC | [42] |
Porous Co3O4 | Decomposition of VOC | [43] | ||
3D layered LiCoO2 | OER | [44] | ||
Gram-scale spinel Co3O4 nanoparticles | OER | [45] | ||
LFP | Fe-N-P-doped catalysts | ORR | [48] | |
Defect-rich NiFe catalysts | OER | [49] | ||
NCM | Mn-based multi-oxide catalysts | Decomposition of VOC | [53] | |
Ternary NCM electrocatalyst | OER | [54] | ||
NiMnCo-based catalysts | OER/ORR | [55] | ||
Anode | C | N-Fe-doped carbon-based catalysts | ORR | [58] |
Cu/C | CuO/C catalyst | Decomposition of VOC | [59] | |
Mix | LCO/LFP | CoFe/C catalysts | ORR | [60] |
LCO/C | Co@NG catalysts | ORR | [61] |
Spent LiCoO2
Li1-xMO2, represented by the LiCoO2 with layered structure, was the primary commercial cathode material (CM) in the early era of LIBs[41]. Its extensive use in portable electronic devices stemmed from its outstanding overall performance and high volumetric energy density. However, with the growth and widespread adoption of these devices, a substantial number of spent LIBs with LiCoO2 cathodes are generated annually. The component of Co possesses physiological toxicity and is a typical heavy metal pollutant. Additionally, Co is a crucial metal resource, and Co-based catalysts exhibit excellent catalytic properties applicable in various catalytic reaction systems. Consequently, the recycling of spent LiCoO2 has garnered significant attention in recent years.
Co-based catalysts are recognized for their robust catalytic oxidation properties, making them highly effective in degrading harmful organic compounds. Zhao et al. recovered the LiCoO2 cathode through a meticulous calcination process [Figure 2A][42]. They utilized the resulting CM, composed of 94% LiCoO2 and 6% Co3O4 and other constituents, directly as a catalyst for activating peroxymonosulfate (PS) in degrading the levofloxacin hydrochloride (LFX) within wastewater. The catalytic mechanism within the CM catalyst was elucidated, highlighting the pivotal role of the Co2+/Co3+ species in PS activation, while the SO4- group and non-radioactive singlet oxygen in PS were identified as key contributors to the LFX degradation process. Upon careful optimization of the degradation conditions, a high LFX removal rate of 94% was achieved. Hossain et al. developed thermally isolation and controlled recycling methods for selectively regenerating spent LiCoO2 cathodes[43]. Through this process, they transformed the LiCoO2 into porous
Figure 2. (A) Schematic diagram of reclaimed CM as a catalyst for activating PS in the degradation of the LFX within wastewater. Quoted with permission from Zhao et al.[42]; (B) Flow chart for the reconstruction and process of spent LiCoO2. Quoted with permission from Kang et al.[44]. CM: Cathode material; PS: peroxymonosulfate; LFX: levofloxacin hydrochloride; SLCO: spent lithium cobalt oxide; DLSLCO: delithiation from spent lithium cobalt oxide.
Co-based catalysts are also widely acknowledged for their outstanding electrocatalytic properties in oxygen evolution reaction (OER) and oxygen reduction reaction (ORR). Kang et al. recovered spent LiCoO2 cathodes into a three-dimensional layered LiCoO2 catalyst through a two-step chemical delithiation and hydrogen thermal treatment process [Figure 2B][44]. This restructuring created additional active sites and oxygen vacancies within the catalyst, enhancing its electrocatalytic performance. The developed catalyst exhibited remarkable capabilities in promoting charge transfer, significantly enhancing OER performance. The results showcased a low overpotential of 365 mV and a minimal Tafel slope of 67 mV·dec-1 at a current density of 10 mA·cm-2. Kim et al. synthesized gram-scale spinel Co3O4 nanoparticles, serving as OER catalysts, by strategically applying the stepwise leaching reaction method on spent LiCoO2 cathodes[45]. This approach resulted in an increased number of electrocatalytically active sites within the catalyst. Consequently, the Co3O4 nanoparticles demonstrated superior OER catalytic activity, comparable to the widely acknowledged RuO2 catalyst.
In addition, several teams recovered Co from spent LiCoO2 for catalytic pyrolysis of biomass for hydrogen production. Yu et al. employed carbothermal reduction to recover Co from spent LiCoO2 for biomass pyrolysis and steam reforming for H2 production, investigating the impact of various carbon sources on the recovery efficiency[46]. They observed that the energy consumption was relatively low when utilizing coconut shell carbon as the carbon source for the reaction. Furthermore, considering the impacts of pore structure of carbon materials on reduction, they prepared activated carbon with a specific surface area of 653.0 m2·g-1 for Co recovery. By optimizing the mass ratio of the CM to the activated carbon, they achieved a H2 yield of up to 28.3 mmol·g-1.
Spent Li1-xFePO4 (0 < x < 1)
LiFePO4 cathodes, classified as an olivine material, have gained widespread adoption in electric and hybrid vehicles owing to their exceptional thermal stability, extended cycle life, and cost-effectiveness[47]. The rising prevalence of electric vehicles in recent years would lead to a substantial increase in the accumulation of waste LiFePO4 batteries. However, the advantageous characteristic of LiFePO4 is that the Fe element within its composition can be efficiently recovered from these spent batteries. The recovered Fe functions as a valuable resource for producing Fe-based catalysts with commendable physical and chemical stability. These catalysts exhibit low sensitivity to moisture, further enhancing their practical utility, and showcase high catalytic activity, with Fe3+/Fe2+ acting as the primary active component.
Luo et al. conducted a recovery of spent LiFePO4 cathodes using a leaching method, employing H2SO4-H2O2 as the leaching agent [Figure 3A][48]. The resulting FePO4 was subjected to further calcination under a nitrogen atmosphere to generate Fe-N-P-doped carbon-based catalysts tailored for ORR. These Fe-N-P-doped catalysts showcased exceptional ORR performance, particularly in an alkaline medium. Remarkably, the Fe-N-P-doped catalysts demonstrated a high peak power density of up to 80 mW·cm-2 when applied in zinc (Zn)-air batteries. Cui et al. synthesized defect-rich NiFe catalysts by employing a recycling method for spent LiFePO4 cathodes, involving impregnation and combination with Ni [Figure 3B][49]. Theoretical calculations conducted in their study revealed that the incorporated Ni was crucial in optimizing the free energy of the *OOH intermediate. This effectively activated the Fe sites within the catalyst. Additionally, the catalyst benefited from a substantial number of oxygen defects, which facilitated the oxygen desorption. The synergistic effect resulting from the presence of Ni and oxygen defects manifested in the impressive OER catalytic activity of the catalyst. At a current density of 10 mA·cm-2, the obtained catalyst exhibited a low overpotential of 285 mV and a small Tafel slope of 45 mV·dec-2.
Figure 3. (A) Schematic illustration of the recycling process of spent LiFePO4 batteries to prepare Fe-N-P-doped catalysts. Quoted with permission from Luo et al.[48]; (B) Schematic diagram of the recycling process of spent LiFePO4 to regenerate defect-rich NiFe catalysts. Quoted with permission from Cui et al.[49]. LIBs: Lithium-ion batteries; LFP: lithium iron phosphate.
Spent LiNixCoyMn1-x-yO2 (0 < x, y < 1)
The ternary layered transition metal oxide material, LiNixCoyMn1-x-yO2 (NCM), has garnered widespread attention as a promising CM for LIBs[50-52]. This material offers a range of advantages, including low cost, a high specific discharge capacity, excellent cycling performance at both room and high temperatures, and a structurally stable framework. One feature of NCM is its flexibility in composition, allowing for the adjustment of the Ni, Co, and Mn ratios to meet specific requirements. This adaptability results in various forms of NCM electrode materials, each exhibiting distinct properties based on the variation in transition metal elements. Due to its composition of various transition metals, the spent NCM demonstrates high recycling efficiency, making it a valuable resource for producing diverse catalysts. These catalysts can find applications in a wide range of catalytic reactions, showcasing the material’s potential to contribute to various catalytic processes.
Guo et al. employed a citric acid leaching method to efficiently recover precious metals from spent NCM cathodes [Figure 4A][53]. Subsequently, they utilized the recovered materials to synthesize Mn-based multi-oxide catalysts designed for the oxidation of volatile organic compounds (VOCs). The resulting catalysts were characterized by several advantageous features, including a large specific surface area, a rich mesoporous structure, excellent low-temperature reducibility, elevated Mn4+ and lattice oxygen concentration, and a robust weak acid site strength. These distinctive characteristics collectively contributed to the exceptional performance of the obtained catalysts in the oxidation of toluene, a representative VOC. Li et al. demonstrated an electrochemical approach by applying electrochemical delithiation technology to directly repurpose spent NCM cathodes into a ternary NCM electrocatalyst for the OER [Figure 4B][54]. This electrochemical delithiation process induced significant alterations in the electronic and microstructure of the catalyst. As a result, a substantial number of Ni3+ and oxygen vacancies were formed. These modifications not only enhanced the electronic conductivity of the catalyst but also provided an increased number of active sites for OER. The optimized NCM electrocatalyst exhibited good performance, characterized by a low overpotential of 270 mV at 10 mA·cm-2. Furthermore, the catalyst demonstrated excellent stability, showcasing durability over a period of 300 h. Jiao et al. repurposed waste NCM cathodes, converting them into highly efficient NiMnCo-based catalysts by combining acid leaching and radiant heating techniques [Figure 4C][55]. These catalysts were then applied as cathodes in zinc-air batteries. The synthesized catalyst showcased a core-shell structure featuring face-centered cubic Ni in the core and spinel NiMnCoO4 in the shell. This unique architecture led to a redistribution of the electronic structure of the prepared catalyst, resulting in a lowered energy barrier for the ORR and OER processes, ensuring high electrocatalytic activity. The constructed zinc-air battery exhibited impressive performance metrics, including a high power density of 187.7 mW·cm-2 and long cycle stability with a sustained performance over 200 h at a current density of 10 mA·cm-2.
Figure 4. (A) Flow chart of utilizing the recovered materials from spent ternary LIBs to synthesize Mn-based multi-oxide catalysts. Quoted with permission from Guo et al.[53]; (B) Schematic diagram of reuse of spent LiNi0.94Co0.05Mn0.01O2 cathodes via electrochemical delithiation technology. Quoted with permission from Li et al.[54]; (C) Schematic diagram of preparation for NiMnCo-based catalysts from spent LIBs. Quoted with permission from Jiao et al.[55]. LIBs: Lithium-ion batteries; NCM: LiNixCoyMn1-x-yO2; de: delithiation; AC: activated carbon.
It is noteworthy to mention that, alongside the primary focus on LIB recycling, recycling other types of spent rechargeable batteries, such as nickel-metal hydride (Ni-MH) batteries, can also result in the recovery of valuable materials. For instance, Pham et al. demonstrated the recycling of the cathode of spent Ni-MH batteries to produce NiO-based electrodes for use in supercapacitors, showcasing a maximum capacitance of 106 C·g-1 at 0.5 A·g-1[56]. Furthermore, they developed the LiNi0.5Mn1.5O4 cathode applied in LIBs with a discharge specific capacity of 137.4 mAh·g-1.
Overall, the recycling strategy for recovering spent LIB cathodes to prepare catalysts offers a dual benefit by preventing the wastage of precious metals such as Ni, Co, and Mn while curbing their environmental pollution. Economically, the catalyst preparation process involves high-temperature calcination, entailing some energy consumption. Nonetheless, when factoring in the catalytic efficiency and energy-saving attributes during catalytic reactions, catalysts derived from recycled cathodes yield higher economic value than the energy expended in their preparation.
Recycling of anodes
Graphite is presently the preferred anode material in LIBs, primarily due to its excellent electrochemical properties[57]. However, recycling graphite from spent LIBs has faced challenges, primarily associated with its relatively low added value and the difficulty in recovery processes. Despite its prevalence in battery technology, graphite recycling has not been as extensively pursued as other components of LIBs. Recently, there has been a growing awareness of the environmental impact and economic potential associated with recycling anode materials, including graphite and Cu current collectors, from spent LIBs [Table 1]. The surge in the number of waste LIBs, coupled with the widespread use of graphite active materials and Cu in various fields, such as catalysis, has underscored the need for developing effective recycling strategies.
Ruan et al. conducted a study wherein they repurposed graphite anodes extracted from spent LIBs
Figure 5. (A) Schematic illustration of the preparation of N- and Fe-doped carbon-based catalysts recovered from spent LIBs. Quoted with permission from Ruan et al.[58]; (B) Schematic diagram of reaction mechanism of CuO/C catalyst recovered from spent LIBs to activate PS for RhB degradation. Quoted with permission from Zhao et al.[59]. LIBs: Lithium-ion batteries; PS: peroxymonosulfate; RhB: Rhodamine B.
Compared to cathode recovery, the process of recovering anodes for catalyst preparation does not require high ambient temperatures, leading to reduced energy consumption and frequently yielding catalysts with notable catalytic performance. Nevertheless, since anode materials such as C and Cu are more prevalent compared to cathodes, the economic advantages of utilizing recovered anodes for catalyst production are relatively modest.
Synergistic recycling of cathodes and anodes
Recycling various cathodes and anodes sourced from diverse spent LIBs has the potential to generate a versatile array of catalytic materials. This includes the production of metal-doped catalysts and/or carbon-based transition metal-doped catalysts. The resulting recycled materials hold promise for applications across various catalytic fields. For example, Jiao et al. employed a synergistic recycling strategy, utilizing leaching and calcination methods, to selectively extract Co and Fe from spent LiCoO2 and LiFePO4 cathodes, respectively [Figure 6A][60]. Subsequently, they synthesized CoFe/C catalysts utilizing carbon derived from sawdust as a carrier for zinc-air batteries. The study revealed that CoFe nanoparticles were uniformly dispersed on the carbon carrier, attributed to electrostatic interactions between Co3+ and Fe3+. The distinctive electronic structure of the catalyst contributed to its exceptional ORR performance. In practical battery applications, the cathode employing CoFe/C showcased an extended cycle stability of 350 h and a high power density of nearly 200 mW·cm-2. Serbara Bejigo et al. employed hydrometallurgy to recover Co metal from spent LiCoO2 batteries, and concurrently, graphite anodes were also reclaimed and transformed into graphene oxide [Figure 6B][61]. These retrieved components were then integrated through a calcination method to synthesize cobalt-integrated nitrogen-doped graphene (Co@NG) catalysts, specifically designed for the ORR. The obtained Co@NG exhibited noteworthy activity in ORR, demonstrating performance comparable to commercial Pt/C catalysts. Moreover, when incorporated into a direct methanol fuel cell setup with Co@NG as the cathode and commercial Pt-Ru/C as the anode, the fuel cell exhibited exceptional performance and sustained stability.
Figure 6. (A) Schematic illustration of the CoFe/C catalyst cathode synthesis from spent LIBs to optimize Zn-air batteries. Quoted with permission from Jiao et al.[60]; (B) The route of recycling spent LIBs to prepare Co@NG catalyst. Quoted with permission from Serbara Bejigo et al.[61]. LIBs: Lithium-ion batteries.
ADVANCED SPECTROSCOPIC CHARACTERIZATIONS FOR FUNDAMENTAL INSIGHT INTO CATALYSTS
Catalyst characterization is pivotal in the entire catalyst lifecycle, from preparation to application. This process allows for the feedback and prediction of catalyst properties, facilitating the targeted design of catalysts and the exploration of catalytic reaction mechanisms. Comprehensive catalyst characterization encompasses both macroscopic and microscopic perspectives. Macroscopic characterization methods in catalysis primarily concentrate on factors such as catalyst density, particle size, pore structure, pore size distribution, specific surface area, and thermal and pressure resistance. These macroscopic attributes provide crucial insights into the physical and mechanical properties of the catalyst, influencing its performance under various conditions. On the microscopic level, catalyst characterization delves into studying the catalyst surface and/or interface. This includes analyzing the component distribution, active center, surface structure, grain size, coordination environment, energy state, and redox properties. These microscopic details are vital for understanding the intricacies of catalyst behavior at the atomic and molecular levels, allowing for a deeper comprehension of catalytic processes. On the other hand, the significance of in situ characterization becomes increasingly apparent as a pivotal aspect in capturing dynamic information essential for understanding catalytic reactions. The real-time insights afforded by in situ characterization are decisive in evaluating catalyst performance and unraveling the intricate details of catalytic mechanisms. Herein, we highlight X-ray-based techniques and in situ surface-sensitive enhanced Raman techniques as powerful tools that address some of the most critical and fundamental questions encountered in exploring catalytic reaction mechanisms.
X-ray-based techniques
X-ray, characterized by short wavelengths and high energy, interacts with the analyte to provide valuable insights into its structure and composition. Numerous X-ray-based analysis methods have been established, including X-ray absorption, X-ray diffraction (XRD), and X-ray photoelectron spectroscopy (XPS), leveraging the unique characteristics of X-ray. These techniques yield rich chemical information about the sample being analyzed, encompassing elemental composition, content, valence, and chemical bonding. Additionally, they provide insights into the structure, surrounding environment, and depth distribution of the sample. X-ray-based analysis serves as a potent tool for characterizing catalysts derived from recycled lithium-ion cathodes, enabling the exploration of their structure, chemical components, catalytic performance, and catalytic mechanisms.
X-ray absorption spectroscopy
X-ray absorption spectroscopy (XAS) employs the energy of X-ray photons as a variable to excite photoelectrons on a solid surface. These photoelectrons are then analyzed with an energy analyzer to determine the X-ray absorption coefficient of the material. XAS offers valuable insights into the coordination environment and chemical state of the probed atoms, making it a common technique for characterizing various catalyst types, including single-atom catalysts, surface reactions, nanoscale catalysts, and atomic occupancy.
XAS is typically divided into two regions: X-ray absorption near edge structure (XANES) and extended
Figure 7. XAS characterization of catalysts prepared from recycled LIBs. (A) and (B) XANES spectra of the CoFe/C catalyst and the respective metal foils; (C) and (D) k2-weighted R-space EXAFS spectra of the CoFe/C catalyst and the respective metal foils. Quoted with permission from Jiao et al.[60]. XAS: X-ray absorption spectroscopy; LIBs: lithium-ion batteries; XANES: X-ray absorption near edge structure; EXAFS: extended X-ray absorption fine structure.
X-ray diffraction and X-ray photoelectron spectroscopy
XRD analysis serves to identify the composition of a material and scrutinize its internal crystal structure. When X-rays are irradiated into a sample from various angles, they undergo diffraction, causing a change in the diffraction pattern corresponding to the crystal structure, leading to deflection. By scrutinizing the direction and intensity of this deflection and applying the Bragg equation to interpret the signal output, insights into the internal crystal structure of the sample can be gleaned. Zhou et al. employed a pH gradient method to recycle waste LiMn2O4 from spent LIBs[62]. The process involved leaching the spent LiMn2O4 cathodes and subsequently calcining the resulting metal oxides to obtain the desired materials for synthesizing the OER catalyst. Experimental optimization was conducted to enhance the metal leaching rate by adjusting parameters such as leaching current, temperature, and time. These variables were subjected to gradient experiments, and the resulting products were characterized using XRD to determine the optimal experimental conditions. XPS employs X-ray with a specific energy to irradiate a sample, resulting in the release of electrons from the atoms of the sample being measured, thus generating free electrons. By analyzing the energies of the excited photoelectrons, XPS enables investigation into the composition of elements in the catalyst, its chemical state, and other relevant properties. Zhao et al. synthesized catalysts by repurposing LCO anodes to activate PS in degrading LFX within wastewater[42]. XPS results revealed the co-existence of Co2+ and Co3+ species, which played a significant role in activating PS.
Raman spectroscopy
Raman spectroscopy stands out as a straightforward, efficient, and non-destructive analysis method, enabling direct application for testing target samples[63,64]. Its efficacy becomes evident in exploring and identifying intermediates during reactions, offering valuable insights into molecular structures and chemical bonds while preserving the sample integrity. For example, Wang et al. repurposed graphite anodes from spent LIBs and synthesized reduced graphene oxide (rGO) used for catalytic ozone oxidation. The catalyst structure was characterized by Raman spectroscopy, revealing that the obtained rGO exhibited a higher
Surface-enhanced Raman spectroscopic strategy
Surface-enhanced Raman spectroscopy (SERS) is a technique that leverages nanostructures with surface plasmon resonance (SPR) effects to amplify Raman signals by up to 6~10 orders of magnitude[64,67,68]. This enhancement is achieved by concentrating the electromagnetic field into a localized space[69,70]. SERS goes beyond offering chemical fingerprints of samples. It can detect molecules at the single-molecule level and provides high spatial resolution. Over the past two decades, SERS technology has experienced substantial development in both fundamental and applied research directions, including catalytic fields. Nonetheless, the robust SERS effects are primarily associated with nanostructured coinage metals such as Au, Ag, and Cu[64,71]. The practical utility of conventional SERS has been constrained by the choice of substrate material and its structure. Consequently, there have been endeavors in the literature to overcome these limitations by borrowing SPR strategies. These efforts aim to extend the applicability of SERS to non-SERS active substrates, marking a significant advancement in broadening the scope of SERS applications.
For example, Yang et al. engineered bifunctional Au@CdS core-shell structured nanoparticles, strategically applied onto an Au substrate [Figure 8A][72]. This combined substrate not only serves as SPR-enhanced photocatalysts for efficiently degrading organic compounds such as methylene blue and p-nitrophenol but also functions as a SERS substrate allowing the real-time monitoring of the reaction process and probing the intricacies of the reaction mechanism. Ze et al. conducted an in situ electrochemical Raman spectroscopic study of the ORR process catalyzed by PtNi alloy catalysts, employing a borrowing SERS strategy[73]. They synthesized Au@PtNi nanoparticles by coating PtNi catalysts onto the surface of Au nanoparticles [Figure 8B]. The Raman signal amplification was achieved by leveraging the SERS effect of the nanostructured Au. Their findings revealed that increasing the Ni content in PtNi enhanced the binding of
Figure 8. (A) Au@CdS core-shell catalyst used for SPR enhanced photocatalytic degration. Quoted with permission from Yang et al.[72]; (B) Schematic illustration of investigation of the crucial role of PtNi alloy catalysts for enhancing ORR by borrowing SERS strategy. Quoted with permission from Ze et al.[73]. SPR: Surface plasmon resonance; ORR: oxygen reduction reaction; SERS: surface-enhanced Raman spectroscopy.
Shell-isolated nanoparticle-enhanced Raman spectroscopic strategy
As a derivative of SERS, shell-isolated nanoparticle (SHIN)-enhanced Raman spectroscopy (SHINERS), developed by our group in 2010, has progressively evolved into a fundamental technology in the realm of enhanced Raman spectroscopy[74,75]. This innovative technique involves using SHINs, where the metal nanoparticle cores (typically Au or Ag) with inherent SERS activity are enveloped by an ultra-thin, pinhole-free, and chemically inert oxide shell (such as SiO2 or Al2O3). The presence of this inert shell serves to prevent direct contact between the internal SERS active source and the external chemical environment, ensuring a controlled and isolated environment[76,77]. Importantly, the ultrathin shell does not compromise the Raman signal enhancement. These distinctive features of SHINERS address critical limitations present in traditional SERS and meet the requirements of in situ monitoring of electrocatalytic and multiphase catalytic processes in diverse environments, making SHINERS widely used in various catalytic fields.
Dong et al. conducted a thorough exploration of the ORR process on the surface of Pt(hkl) single crystals using the in situ SHINERS technique [Figure 9A][77]. Their study successfully captured direct Raman spectral evidence of crucial reactive intermediate species, including O2-, OH*, and HO2*, which was further confirmed by isotopic substitution experiments and theoretical calculations [Figure 9B]. This comprehensive approach enabled the proposal of a mechanistic understanding of the ORR on the surface of Pt(hkl) single crystals under acidic conditions. The combination of experimental evidence and theoretical insights provided a nuanced perspective on the intricacies of the catalytic processes at the atomic level. Zhang et al. delved into the ORR mechanism within Na-O2 cells using in situ SHINERS techniques[78]. They meticulously investigated the aprotic Na+-ORR process on the surfaces of Au(hkl) single crystals. Their detailed exploration yielded direct spectroscopic evidence of superoxide on Au(110) and peroxide on Au(100) and Au(111) as intermediates or products, unraveling the molecular-level intricacies of the reaction mechanism. The combined findings from experimental results and theoretical simulation analyses led to the conclusion that the surface effects of the Au(hkl) electrode on aprotic Na+-ORR activity are primarily attributed to the distinct adsorption behaviors of Na+ and O2.
Figure 9. (A) Schematic diagram of the investigation of oxygen reduction reaction process on Pt(hkl) using SHINERS; (B) The proposed mechanism of oxygen reduction reaction on Pt(hkl) surfaces. Quoted with permission from Dong et al.[77]. SHINERS: Shell-isolated nanoparticle-enhanced Raman spectroscopy.
SHINERS-gap and SHINERS-satellite strategies
Despite the notable success of SHINERS in facilitating in situ studies of electrocatalytic processes, particularly on single-crystal model electrodes, challenges arise when probing interfacial processes on real nanocatalysts. The inherent limitations stem from the small size of actual nanocatalyst particles and the associated weak Raman enhancement. In response to these challenges, our group has pioneered the development of SHINERS-gap and SHINRES-satellite strategies, building upon the foundation of the SHINERS technique. These innovative strategies aim to overcome the constraints posed by actual nanocatalysts, thereby extending the applicability of SHINERS to real-world catalytic systems.
Wei et al. introduced an innovative SHINER-gap strategy, building upon the SHIN/nanocatalyst/SiO2/Au structure [Figure 10A][79]. This approach facilitated an in situ investigation of structure-activity relationships concerning the selective oxidation of benzyl alcohol over Au@Pd core-shell nanocatalysts [Figure 10B]. The results revealed that the tensile strain effect induced by the Pd shell was pivotal in promoting oxygen activation. Importantly, the selective oxidation activity of the catalyst for benzyl alcohol exhibited a notable increase at an optimal shell thickness [Figure 10C and D]. Zhang et al. established a SHINERS-satellite structure by assembling nanocatalysts, such as PtFe and Pd, onto the surface of Au@SiO2 SHINs
Figure 10. (A) Schematic diagram of the SHINERS-gap strategy; (B) Theoretical simulation of the SHINERS-gap configuration; (C) and (D) Morphologies of SHIN and SHINERS-gap configuration. Quoted with permission from Wei et al.[79]. SHINERS: Shell-isolated nanoparticle-enhanced Raman spectroscopy.
Figure 11. (A) Schematic diagram of the SHINERS-satellite strategy; (B) Schematic diagram of CO oxidation on SHINERS-satellite configuration; (C) Theoretical simulation of the SHINERS-gap configuration. Quoted with permission from Zhang et al.[80]. SHINERS: Shell-isolated nanoparticle-enhanced Raman spectroscopy.
CONCLUSION AND OUTLOOK
To summarize, we started by providing a brief overview of the recycling processes of spent LIBs and outlining the interconnectedness between battery recycling and catalysis. Notably, the midstream and downstream stages in the recycling stream of spent LIBs exhibit a significant association with catalysis. We then focused on three catalytic strategies within hydrometallurgy techniques for improving the recycling rate and efficiency of the spent LIBs and discussed transforming reclaimed valuable materials into catalysts tailored to diverse catalytic applications. Moreover, we underscored the pivotal role of advanced spectroscopic characterizations, particularly surface-sensitive enhanced Raman techniques, in unraveling the intricate reaction mechanisms of both model and practical catalysts at the molecular level.
To explore the full potential of catalysis in recycling spent LIBs and harness the capabilities of advanced characterization techniques in studying catalytic reactions, several practical suggestions for future development are outlined below: (1) Currently, diverse catalytic methods have been developed with broad applicability and demonstrated efficacy in various leaching systems. To further enhance leaching efficiency, future emphasis can be placed on optimizing the pretreatment process for electrode materials. For instance, applying Joule-heat technology would enable the rapid carbothermal reduction of high valence metal compounds in a matter of seconds. This rapid reduction can substantially enhance the solubility of these compounds in the solvent, significantly improving the metal leaching rate. Furthermore, achieving green standards is imperative for the leaching system. Deep eutectic solvents (DES), marked by low cost, non-toxicity, and reusability, deserve heightened consideration. Given the vast diversity within DES systems, they are anticipated to emerge as a significant and versatile option for hydrometallurgical processes; (2) The original metal salts can be obtained through conventional pyrometallurgy and hydrometallurgy, serving as catalyst precursors or resynthesized electrode materials. However, there is a pressing need to enhance the recovery process to achieve greater energy conservation and emission reduction. It is crucial to note that any recycling method that compromises the energy storage structure is not the most energy-efficient. There is growing optimism surrounding the emerging direct recycling method, positioning it as the primary direction for environmentally conscious and energy-efficient recycling practices; (3) The purity of the catalyst precursor significantly influences its performance, with higher purity correlating to more favorable conditions for directed synthesis. For instance, refining recovered catalysts through electrometallurgy offers distinct advantages, including heightened efficiency, reduced costs, and enhanced product purity. Through meticulous control of current potential, considerable purification of precipitates can be achieved. Notably, despite its potential, this purification method remains underutilized, emphasizing its significance as a promising avenue for future endeavors in catalyst recovery from spent LIBs; (4) In situ characterization techniques are advancing to offer researchers more robust information on catalytic reactions. However, these techniques may not be ideal for practical catalytic characterization due to the inherent complexities and information-rich nature of the actual working environment. Operando characterization holds the potential to comprehensively reflect the catalytic process in a real-world scenario. The future development of operando characterization techniques can delve into the actual state of catalytic reactions, providing significant insights into catalyst reaction mechanisms, the practical catalytic environment, and failure mechanisms.
DECLARATIONS
Authors’ contributions
Prepared and revised the manuscript: Sun HL, Gu Y, Xu YP, Ding L
Designed and revised the manuscript: Gu Y, Li JF
Availability of data and materials
Not applicable.
Financial support and sponsorship
This work was supported by the National Natural Science Foundation of China (T2293692, 21925404, and 22021001).
Conflicts of interest
All authors declared that there are no conflicts of interest.
Ethical approval and consent to participate
Not applicable.
Consent for publication
Not applicable.
Copyright
© The Author(s) 2024.
REFERENCES
1. Sun J, Liu J, Wang Y, Yuan H, Yan Z. Development status, policy, and market mechanisms for battery energy storage in the US, China, Australia, and the UK. J Renew Sustain Ener 2023;15:024101.
2. Koohi-Fayegh S, Rosen MA. A review of energy storage types, applications and recent developments. J Energy Storage 2020;27:101047.
3. Reddy MV, Mauger A, Julien CM, Paolella A, Zaghib K. Brief history of early lithium-battery development. Materials 2020;13:1884.
4. Feng T, Guo W, Li Q, Meng Z, Liang W. Life cycle assessment of lithium nickel cobalt manganese oxide batteries and lithium iron phosphate batteries for electric vehicles in China. J Energy Storage 2022;52:104767.
5. Mejame PPM, Jung DY, Lee H, Lee DS, Lim SR. Effect of technological developments for smartphone lithium battery on metal-derived resource depletion and toxicity potentials. Resour Conserv Recy 2020;158:104797.
6. Lang P, Yuan N, Jiang Q, Zhang Y, Tang J. Recent advances and prospects of metal-based catalysts for oxygen reduction reaction. Energy Technol 2020;8:1900984.
7. Chen X, Liu J, Yuan T, et al. Recent advances in earth-abundant first-row transition metal (Fe, Co and Ni)-based electrocatalysts for the oxygen evolution reaction. Energy Mater 2022;2:200028.
8. Kumar J, Neiber RR, Park J, et al. Recent progress in sustainable recycling of LiFePO4-type lithium-ion batteries: strategies for highly selective lithium recovery. Chem Eng J 2022;431:133993.
9. Bhatt A, Tiwari S, Ongsakul W. A review on re-utilization of electric vehicle’s retired batteries. In: 2018 International Conference and Utility Exhibition on Green Energy for Sustainable Development (ICUE); 2018 Oct 24-26; Phuket, Thailand. IEEE; 2018. p. 1-5.
10. Lv Y, Luo W, Mo Y, Zhang G. Investigation on the thermo-electric-electrochemical characteristics of retired LFP batteries for echelon applications. RSC Adv 2022;12:14127-36.
11. Zhou M, Li B, Li J, Xu Z. Pyrometallurgical technology in the recycling of a spent lithium ion battery: evolution and the challenge. ACS EST Eng 2021;1:1369-82.
12. Ma Y, Tang J, Wanaldi R, et al. A promising selective recovery process of valuable metals from spent lithium ion batteries via reduction roasting and ammonia leaching. J Hazard Mater 2021;402:123491.
13. Lin J, Li L, Fan E, et al. Conversion mechanisms of selective extraction of lithium from spent lithium-ion batteries by sulfation roasting. ACS Appl Mater Interfaces 2020;12:18482-9.
14. Li J, Wang L, Li L, Lv C, Zatovsky IV, Han W. Metal sulfides@carbon microfiber networks for boosting lithium ion/sodium ion storage via a general metal-aspergillus niger bioleaching strategy. ACS Appl Mater Interfaces 2019;11:8072-80.
15. Chang X, Fan M, Yuan B, et al. Potential controllable redox couple for mild and efficient lithium recovery from spent batteries. Angew Chem Int Ed Engl 2023;62:e202310435.
16. Kong Y, Yuan L, Liao Y, Shao Y, Hao S, Huang Y. Efficient separation and selective Li recycling of spent LiFePO4 cathode. Energy Mater 2023;3:300053.
17. Liang Z, Cai C, Peng G, et al. Hydrometallurgical recovery of spent lithium ion batteries: environmental strategies and sustainability evaluation. ACS Sustain Chem Eng 2021;9:5750-67.
18. Jiang G, Zhang Y, Meng Q, et al. Direct regeneration of LiNi0.5Co0.2Mn0.3O2 cathode from spent lithium-ion batteries by the molten salts method. ACS Sustain Chem Eng 2020;8:18138-47.
19. Ji G, Wang J, Liang Z, et al. Direct regeneration of degraded lithium-ion battery cathodes with a multifunctional organic lithium salt. Nat Commun 2023;14:584.
20. Ma J, Wang J, Jia K, et al. Adaptable eutectic salt for the direct recycling of highly degraded layer cathodes. J Am Chem Soc 2022;144:20306-14.
21. Shi Y, Zhang M, Meng YS, Chen Z. Ambient-pressure relithiation of degraded LixNi0.5Co0.2Mn0.3O2 (0 < x < 1) via eutectic solutions for direct regeneration of lithium-ion battery cathodes. Adv Energy Mater 2019;9:1900454.
22. Wang J, Ma J, Jia K, et al. Efficient extraction of lithium from anode for direct regeneration of cathode materials of spent Li-ion batteries. ACS Energy Lett 2022;7:2816-24.
23. Wang J, Zhang Q, Sheng J, et al. Direct and green repairing of degraded LiCoO2 for reuse in lithium-ion batteries. Natl Sci Rev 2022;9:nwac097.
24. Xu P, Tan DHS, Jiao B, Gao H, Yu X, Chen Z. A materials perspective on direct recycling of lithium-ion batteries: principles, challenges and opportunities. Adv Funct Mater 2023;33:2213168.
25. Li P, Luo S, Zhang L, et al. Progress, challenges, and prospects of spent lithium-ion batteries recycling: a review. J Energy Chem 2024;89:144-71.
26. Fan E, Li L, Wang Z, et al. Sustainable recycling technology for Li-ion batteries and beyond: challenges and future prospects. Chem Rev 2020;120:7020-63.
27. Ji H, Wang J, Ma J, Cheng HM, Zhou G. Fundamentals, status and challenges of direct recycling technologies for lithium ion batteries. Chem Soc Rev 2023;52:8194-244.
28. Makuza B, Tian Q, Guo X, Chattopadhyay K, Yu D. Pyrometallurgical options for recycling spent lithium-ion batteries: a comprehensive review. J Power Sources 2021;491:229622.
29. Niu B, Xu Z, Xiao J, Qin Y. Recycling hazardous and valuable electrolyte in spent lithium-ion batteries: urgency, progress, challenge, and viable approach. Chem Rev 2023;123:8718-35.
30. Zhang X, Li L, Fan E, et al. Toward sustainable and systematic recycling of spent rechargeable batteries. Chem Soc Rev 2018;47:7239-302.
31. Xiong L, Tang J. Strategies and challenges on selectivity of photocatalytic oxidation of organic substances. Adv Energy Mater 2021;11:2003216.
32. Zhang C, Bai L, Chen M, et al. Modulating the site density of mo single atoms to catch adventitious O atoms for efficient H2O2 oxidation with light. Adv Mater 2023;35:2208704.
33. Lv X, Lin J, Huang Q, et al. An emerging and consummate photocatalysis-assisted strategy for efficient recycling of spent lithium-ion batteries. ACS Energy Lett 2023;8:4287-95.
35. Jiang F, Chen Y, Ju S, et al. Ultrasound-assisted leaching of cobalt and lithium from spent lithium-ion batteries. Ultrason Sonochem 2018;48:88-95.
36. Li L, Zhai L, Zhang X, et al. Recovery of valuable metals from spent lithium-ion batteries by ultrasonic-assisted leaching process. J Power Sources 2014;262:380-5.
37. Li H, Berbille A, Zhao X, Wang Z, Tang W, Wang ZL. A contact-electro-catalytic cathode recycling method for spent lithium-ion batteries. Nat Energy 2023;8:1137-44.
38. Zhang YC, Yu WH, Xu SM. Enhanced leaching of metals from spent lithium-ion batteries by catalytic carbothermic reduction. Rare Met 2023;42:2688-99.
39. Nshizirungu T, Rana M, Jo YT, Park JH. Rapid leaching and recovery of valuable metals from spent lithium ion batteries (LIBs) via environmentally benign subcritical nickel-containing water over chlorinated polyvinyl chloride. J Hazard Mater 2020;396:122667.
40. Porvali A, Chernyaev A, Shukla S, Lundström M. Lithium ion battery active material dissolution kinetics in Fe(II)/Fe(III) catalyzed Cu-H2SO4 leaching system. Sep Purif Technol 2020;236:116305.
41. Lyu Y, Wu X, Wang K, et al. An overview on the advances of LiCoO2 cathodes for lithium-ion batteries. Adv Energy Mater 2021;11:2000982.
42. Zhao Y, Yuan X, Jiang L, Li X, Zhang J, Wang H. Reutilization of cathode material from spent batteries as a heterogeneous catalyst to remove antibiotics in wastewater via peroxymonosulfate activation. Chem Eng J 2020;400:125903.
43. Hossain R, Sahajwalla V. Microrecycled Co3O4 from waste lithium-ion battery: synthesis, characterisation and implication in environmental application. J Environ Chem Eng 2022;10:107858.
44. Kang J, Tang D, Liu Y, et al. Hydrogen-treated spent lithium cobalt oxide as an efficient electrocatalyst for oxygen evolution. Ind Eng Chem Res 2023;62:3882-8.
45. Kim J, Kim HG, Kim HS, Dang Van C, Lee MH, Jeon KW. Facile gram-scale synthesis of Co3O4 nanocrystal from spent lithium ion batteries and its electrocatalytic application toward oxygen evolution reaction. Nanomaterials 2022;13:125.
46. Yu Y, Li X, Shao S, Zhang P, Jiang J. Hydrogen production via biomass fast pyrolysis and in-line steam reforming using carbon reduced cathode material of spent LiCoO2 batteries as catalyst. Fuel 2024;357:129659.
47. Wang J, Sun X. Olivine LiFePO4: the remaining challenges for future energy storage. Energy Environ Sci 2015;8:1110-38.
48. Luo K, Zhou M, Liu T, et al. A high-performance zinc-air battery cathode catalyst from recycling of spent lithium iron phosphate batteries. Small Struct 2023;4:2300107.
49. Cui B, Liu C, Zhang J, et al. Waste to wealth: defect-rich Ni-incorporated spent LiFePO4 for efficient oxygen evolution reaction. Sci China Mater 2021;64:2710-8.
50. Zhang SS. Problems and their origins of Ni-rich layered oxide cathode materials. Energy Storage Mater 2020;24:247-54.
51. Zhao G, Gong ZL, Li YX, Yang Y. Study on surface modification of LiNi0.96Co0.02Mn0.02O2 with tungsten oxide and phosphotungstic acid. J Electrochem 2022;29:2204281.
52. Li Z, Huang X, Liang J, et al. Element doping induced microstructural engineering enhancing the lithium storage performance of high-nickel layered cathodes. J Energy Chem 2023;77:461-8.
53. Guo M, Li K, Liu L, et al. Manganese-based multi-oxide derived from spent ternary lithium-ions batteries as high-efficient catalyst for VOCs oxidation. J Hazard Mater 2019;380:120905.
54. Li S, Zhu X, Wang X, et al. High-valence Ni3+ construction and stability by electrochemical de-lithiation boosting oxygen evolution. Mater Chem Front 2023;7:5868-78.
55. Jiao M, Zhang Q, Ye C, et al. Recycling spent LiNi1-x-yMnxCoyO2 cathodes to bifunctional NiMnCo catalysts for zinc-air batteries. Proc Natl Acad Sci U S A 2022;119:e2202202119.
56. Pham HD, Krishnan SG, Wang T, et al. Upcycling of nickel oxide from spent Ni-MH batteries as ultra-high capacity and stable Li-based energy storage devices. Sustain Mater Technol 2023;36:e00602.
58. Ruan D, Zou K, Du K, et al. Recycling of graphite anode from spent lithium-ion batteries for preparing Fe-N-doped carbon ORR catalyst. ChemCatChem 2021;13:2025-33.
59. Zhao Y, Wang H, Li X, Yuan X, Jiang L, Chen X. Recovery of CuO/C catalyst from spent anode material in battery to activate peroxymonosulfate for refractory organic contaminants degradation. J Hazard Mater 2021;420:126552.
60. Jiao M, Zhang Q, Ye C, et al. Isolating contiguous Fe atoms by forming a Co-Fe intermetallic catalyst from spent lithium-ion batteries to regulate activity for zinc-air batteries. ACS Nano 2022;16:13223-31.
61. Serbara Bejigo K, Bhunia K, Kim J, Lee C, Back S, Kim SJ. Upcycling end of lithium cobalt oxide batteries to electrocatalyst for oxygen reduction reaction in direct methanol fuel cell via sustainable approach. J Energy Chem 2023;82:148-57.
62. Zhou J, Bing J, Ni J, Wang X, Guan X. Recycling the waste LiMn2O4 of spent Li-ion batteries by pH gradient in neutral water electrolyser. Mater Today Sustain 2022;20:100205.
63. Hess C. New advances in using Raman spectroscopy for the characterization of catalysts and catalytic reactions. Chem Soc Rev 2021;50:3519-64.
64. Tian ZQ, Ren B, Li JF, Yang ZL. Expanding generality of surface-enhanced Raman spectroscopy with borrowing SERS activity strategy. Chem Commun 2007:3514-34.
65. Wang Y, Cao H, Chen L, et al. Tailored synthesis of active reduced graphene oxides from waste graphite: structural defects and pollutant-dependent reactive radicals in aqueous organics decontamination. Appl Catal B Environ 2018;229:71-80.
66. Cowan AJ, Hardwick LJ. Advanced spectroelectrochemical techniques to study electrode interfaces within lithium-ion and lithium-oxygen batteries. Annu Rev Anal Chem 2019;12:323-46.
67. Gu Y, Tang S, Yi J, et al. Nanostructure-based plasmon-enhanced Raman spectroscopic strategies for characterization of the solid–electrolyte interphase: opportunities and challenges. J Phys Chem C 2023;127:13466-77.
68. Ding SY, Yi J, Li JF, et al. Erratum: Nanostructure-based plasmon-enhanced Raman spectroscopy for surface analysis of materials. Nat Rev Mater 2016:16036.
69. Zhang Y, Ze H, Fang P, et al. Shell-isolated nanoparticle-enhanced Raman spectroscopy. Nat Rev Methods Primers 2023;3:37.
70. Wang X, Huang SC, Hu S, Yan S, Ren B. Fundamental understanding and applications of plasmon-enhanced Raman spectroscopy. Nat Rev Phys 2020;2:253-71.
71. Gu Y, Hu YF, Wang WW, et al. An in-situ Raman spectroscopic study on the interfacial process of carbonate-based electrolyte on nanostructured silver electrode. J Electrochem 2023;29:2301261.
72. Yang JL, Xu J, Ren H, et al. In situ SERS study of surface plasmon resonance enhanced photocatalytic reactions using bifunctional Au@CdS core-shell nanocomposites. Nanoscale 2017;9:6254-8.
73. Ze H, Chen X, Wang XT, et al. Molecular insight of the critical role of Ni in Pt-based nanocatalysts for improving the oxygen reduction reaction probed using an in situ SERS borrowing strategy. J Am Chem Soc 2021;143:1318-22.
74. Li JF, Huang YF, Ding Y, et al. Shell-isolated nanoparticle-enhanced Raman spectroscopy. Nature 2010;464:392-5.
75. Li JF, Zhang YJ, Ding SY, Panneerselvam R, Tian ZQ. Core-shell nanoparticle-enhanced Raman spectroscopy. Chem Rev 2017;117:5002-69.
76. Gu Y, You EM, Lin JD, et al. Resolving nanostructure and chemistry of solid-electrolyte interphase on lithium anodes by depth-sensitive plasmon-enhanced Raman spectroscopy. Nat Commun 2023;14:3536.
77. Dong JC, Zhang XG, Briega-Martos V, et al. In situ Raman spectroscopic evidence for oxygen reduction reaction intermediates at platinum single-crystal surfaces. Nat Energy 2019;4:60-7.
78. Zhang J, Zhang XG, Dong JC, et al. Real-time monitoring of surface effects on the oxygen reduction reaction mechanism for aprotic Na-O2 batteries. J Am Chem Soc 2021;143:20049-54.
79. Wei J, Zhang YJ, Qin SN, et al. Understanding the strain effect of Au@Pd nanocatalysts by in situ surface-enhanced Raman spectroscopy. Chem Commun 2019;55:8824-7.
80. Zhang H, Wang C, Sun HL, et al. In situ dynamic tracking of heterogeneous nanocatalytic processes by shell-isolated nanoparticle-enhanced Raman spectroscopy. Nat Commun 2017;8:15447.
Cite This Article
Export citation file: BibTeX | RIS
OAE Style
Sun HL, Xu YP, Ding L, Gu Y, Li JF. Recycling valuable materials from the spent lithium ion batteries to catalysts: methods, applications, and characterization. Chem Synth 2024;4:23. http://dx.doi.org/10.20517/cs.2023.66
AMA Style
Sun HL, Xu YP, Ding L, Gu Y, Li JF. Recycling valuable materials from the spent lithium ion batteries to catalysts: methods, applications, and characterization. Chemical Synthesis. 2024; 4(2): 23. http://dx.doi.org/10.20517/cs.2023.66
Chicago/Turabian Style
Sun, Hao-Long, Yun-Peng Xu, Lian Ding, Yu Gu, Jian-Feng Li. 2024. "Recycling valuable materials from the spent lithium ion batteries to catalysts: methods, applications, and characterization" Chemical Synthesis. 4, no.2: 23. http://dx.doi.org/10.20517/cs.2023.66
ACS Style
Sun, H.L.; Xu Y.P.; Ding L.; Gu Y.; Li J.F. Recycling valuable materials from the spent lithium ion batteries to catalysts: methods, applications, and characterization. Chem. Synth. 2024, 4, 23. http://dx.doi.org/10.20517/cs.2023.66
About This Article
Special Issue
Copyright
Data & Comments
Data
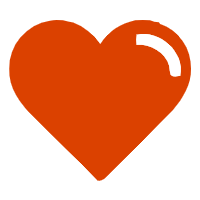

Comments
Comments must be written in English. Spam, offensive content, impersonation, and private information will not be permitted. If any comment is reported and identified as inappropriate content by OAE staff, the comment will be removed without notice. If you have any queries or need any help, please contact us at support@oaepublish.com.