Electrochemical-induced hydrofunctionalizations of alkenes and alkynes
Abstract
The hydrofunctionalizations of readily available alkenes and alkynes are one of the most effective and useful routes to afford diverse value-added compounds. Although traditional hydrofunctionalization strategies catalyzed by metal catalysts present convenient approaches, they are also accompanied by resource consumption and environmental crisis. Electrosynthesis, as a renewable and sustainable technology, has become a cost- and atom-efficient and useful synthetic route. In this review, the electrochemical-induced hydrofunctionalizations of alkenes and alkynes are summarized and presented. In each section, the electrochemical synthetic strategy to access hydrogenation and other hydrofunctionalization (hydroboration, hydrosilylation, hydroalkylation, hydroalkoxylation, hydrocyanation, hydrocarboxylation, etc.) products are elaborated in detail separately. Finally, the current challenges and prospects for electrochemical hydrofunctionalizations of unsaturated carbon‒carbon
Keywords
INTRODUCTION
Alkenes and alkynes, as ubiquitous building blocks, are widely employed in organic synthesis, agricultural chemicals, and biological organic materials[1-3]. Although they can be directly applied, one of the most atomically economical and straightforward routes is hydrofunctionalization addition reactions across multiple bonds[4]. Given the easy accessibility and rich reactivity of alkenes and alkynes, diverse functional groups (FG) have been successfully introduced to construct valuable molecular scaffolds. For the hydrogenation of alkenes and alkynes, plentiful homogeneous[5-7] and heterogeneous[8-10] metal catalytic systems have been explored, involving various earth-abundant metals such as Co[5], Fe[6,7], and Ni[9], along with noble metals such as Pd[8-10], Rh[6,9], and Ru[6]. Moreover, some metal-free systems, such as frustrated Lewis pairs (FLPs)[11], have also made significant progress in catalyzing the hydrogenation of alkenes and alkynes. Similarly, numerous metal catalysts and metal-free systems have also been developed to achieve the other types of hydrofunctionalizations of alkenes and alkynes, such as hydroamination, hydroboration, hydroalkylation, hydrophosphorylation, hydrosilylation, etc. Metals involved in the former include Ag[12], Mn[13], Cu[14,15], Fe[16], Co[17,18], Pd[19], and others[20], while ionic liquids involved in the latter have also been proved to be promising complementary catalysts for the synthesis of hydrogenated products[21-23]. Nevertheless, in this modern era of resource exhaustion and environmental pollution[24], although these catalytic systems have made great progress in the hydrofunctionalizations of alkenes and alkynes, their harsh reaction conditions and hazardous wastes should not be ignored.
Over the past decades, the development of renewable energy sources, such as wind, solar, and biomass, has increased dramatically and tripled the global power generation capacity[25]. This deployment in power generation has driven the transition from the thermochemical to the electrochemical era[26,27]. The conversion of electricity directly to chemical energy offers advantages over the more classic synthetic routes[28-31], which promotes the exciting and ongoing renaissance of electrochemistry since its beginning in the 19th century[32]. As an environmentally friendly and atomically efficient methodology[33], electrochemical synthesis has been involved in the sustainable production of over 35% of value-added compounds[34], including electrochemical manufacturing in the chemical industry[35,36], such as the electrochemical hydrodimerization of acrylonitrile into adiponitrile[37]. The success of electrochemical synthesis depends on its intrinsic properties, in which the chemoselectivity and reactivity of electrochemical reaction can be achieved by adjusting current or potential, avoiding the utilization of hazardous, toxic, and polluting reagents[38,39].
So far, electrochemical transformation has made significant advances through manipulating redox potential[40-43]. In addition to the significant progress made in anodic oxidation reactions[44-46], reductive electrolysis reactions have also received extensive attention in recent years[47]. Compared to traditional approaches, reductive electrolysis provides a promising benign alternative for the hydrofunctionalizations of alkenes and alkynes under mild conditions[48]. The utilization of current allows the addition of H–FG to multiple carbon–carbon (C–C) bonds with low toxicity and high efficiency. Although several impressive reviews regarding the advances of electrochemical reduction have been reported, these reviews mainly summarize the hydrogenation of unsaturated compounds[49,50]. This review [Scheme 1] focuses on the electrochemical hydrofunctionalizations of alkenes and alkynes, highlighting not only the recent advances in their hydrogenation to generate the corresponding alkanes or alkenes.
HYDROFUNCTIONALIZATION REACTIONS
Hydrogenation
Hydrogenation of alkenes and alkynes, which is the introduction of H atoms to π bonds, is widely used from basic research to industrial application. So far, various hydrogenation platforms have been explored and play an irreplaceable role in synthesizing fine chemicals and pharmaceuticals[13,51,52]. Moreover, two employed strategies are summarized. Thereinto, transfer hydrogenation with a non-hydrogen gas (H2) reagent as a hydrogen source represents a more convenient, safer, and more powerful method in preparing hydrogenated products than direct hydrogenation with H2[53]. The electrochemical process, using electrons as redox regents, provides an attractive protocol for the selective transfer hydrogenation of unsaturated hydrocarbons with non-H2 reagents as hydrogen sources[49,50,54,55].
Hydrogenation of alkenes
Hydrogenation of alkenes to afford corresponding alkanes is a fundamental and powerful reaction[56]. So far, some innovative examples induced by electrochemistry have been developed in the presence of various hydrogen transfer reagents, including alcohol, CH3CN, dimethyl sulfoxide (DMSO), H2O, ammonia (NH3), sulfonic acid, and other hydrogen sources.
Hydrogenation of activated alkenes
Generally, unactivated alkenes possess a high reduction potential and are difficult to reduce. Nevertheless, the electron-withdrawing groups (EWGs) based on the unsaturated C–C bonds of alkenes[57] can make the cathodic reduction more practical in the presence of a hydrogen source. Tomida et al. developed an electrochemical hydrogenation method of activated alkenes substituted by different electron-withdrawing ester groups [Scheme 2], using silica gel-supported sulfonic acid (Si–SO3H, size: 40-63 μm, loading:
Scheme 2. Reduction of activated alkenes using the concept of site isolation. Copyright 2016, Elsevier[58].
In 2019, Huang et al. disclosed a chemoselective 1,4-reduction of α,β-unsaturated ketones under constant current using ammonium chloride (NH4Cl) and MeOH as hydrogen donors [Scheme 3A], realizing the selective hydrogenation of alkenes[59]. The scope and limitation of this electrochemical method are also investigated; the substrates of chalcones derivatives with diverse electronic effects (5-11) and α,β-unsaturated ketones containing heterocycles (12) or alkyl substituents (13, 14) are all smoothly reduced to corresponding alkanes in moderate (52%) to excellent (91%) yields. Unfortunately, this electroreduce method is not suitable for substrates (15, 16) where R’ is substituted by an alkyl group. Furthermore, a series of control experiments are performed, including radical trapping and deuterium labeling. A possible reaction mechanism is proposed in the section below of Scheme 3B, in which solvent DMSO is constantly oxidized at the anode surface and continuously provides electrons for the reduction of all substrates. Meanwhile, α,β-unsaturated ketones are reduced and isomerized to obtain stable benzyl radicals, followed by a multi-step protonation and reduction to obtain the target products.
Scheme 3. Electroreduction of α,β-unsaturated ketones. (A) The scope of substrates; (B) A possible mechanism is proposed. Copyright 2019, Royal Society of Chemistry[59].
Deuterium-labeled pharmaceutical compounds are key diagnostic tools for drug and biomarker discovery studies in terms of action and toxicity information, and they also provide significant information for the biological transport and metabolites of drugs[60]. Therefore, the introduction of isotopic labels with cheaper and more accessible routes is urgently needed. Liu et al. realized the electrochemical deuteration reaction of α,β-unsaturated hydrocarbons using D2O as a deuteration source and a sacrificial reducing reagent under neutral electrolysis conditions[61] [Scheme 4]. The utility of graphite felt electrodes on cathode and anode is critical to ensuring the deuterium rates (as high as 99%), chemoselectivity (yield up to 91% in 2 h), and broad substrate applicability (64 examples). Additionally, gram-scale experiments up to 15 g can also be performed, and the deuterium rate (60% yield) is almost the same as the 0.2 mmol (68% yield). Notably, this protocol can also be applied to synthesize some deuterated pharmaceutical compounds (30-35). The detailed mechanistic studies confirm oxygen precipitated on the anode surface promotes this electroreduction process by adjusting the solution pH.
Scheme 4. Electrochemical deuteration reaction using D2O. Copyright 2020, Wiley-VCH[61]. Red numbers: Content of 100% indicates one D atom on one carbon of products.
The same group first disclosed the electrochemical hydrogenation of alkenes with gaseous NH3 as the proton source directly[62] [Scheme 5A]. The undivided electrolysis device is equipped with two carbon electrodes and connects to a balloon filled with NH3 at room temperature. Compound 36 gives a 73% yield under standard conditions with a Faraday efficiency (FE) of 54%. This electrochemical hydrogenation protocol is tolerant to various substrates (40 examples) substituted by valuable groups, including pyridine nitrogen, sulfide, esters, amides, hydroxyl, and nitrile. A fast stepwise electrochemical reduction mechanism of NH3 is proposed [Scheme 5B]. First, alkenes undergo two rounds of cathode reduction and abstract protons on ammonia, while ammonia experiences anion oxidation to form hydrazine. The intermediate hydrazine has been shown to provide protons under standard conditions by a variety of control experiments, thereby, in turn, reducing alkenes to generate the target products and releasing nitrogen gas (N2).
Scheme 5. Electrochemical hydrogenation of alkenes with NH3. (A) The scope of substrates; (B) A possible mechanism is proposed. Copyright 2019, Wiley-VCH[62].
Subsequently, inspired by the work of Cheng and Xia, a simple method for chemoselective electrochemical hydrogenation of unsaturated C–C bonds with DMSO and H2O as hydrogen donors was developed by Qin et al.[63], generating a series of carbonyl compounds and chain alkanes. This reaction is performed in the absence of metal catalysts and external reducing agents. The substrate scope involves acid, esters, amides, and unactivated alkenes. Moreover, gram-scale experiments were also performed smoothly with desired yields, confirming the potential of this protocol in practical production.
Then, Qin et al. realized the electrochemical-induced hydrogenation of electron-deficient internal alkenes to generate saturated ketones[64], using CH3OH as a single hydrogen donor and KSCN as the anode parallel paired electrolysis reagents [Scheme 6A]. Except for alkyl dithioacetals, enamides, and styrene, a series of electron-deficient internal alkenes (44-51) are compatible with this method in good to excellent yield. The addition of KSCN plays an important role in the efficient and smooth generation of these hydrogenated products. A possible reaction mechanism was outlined in Scheme 6B. The target products can be smoothly obtained by the two successive steps of cathode reduction and protonation of ketene dithioacetals. At the anode surface, KSCN is oxidized to (SCN)2 acting as a parallel paired electrolysis agent, while CH3OH is oxidized to methoxide anion and releases hydrogen atoms.
Scheme 6. Electrochemical-induced hydrogenation of electron-deficient internal alkenes. (A) The scope of substrates; (B) A plausible reaction mechanism is proposed. Copyright 2021, Wiley-VCH[64].
In the same year, using fumarate esters as model objects, Derosa et al. [Scheme 7A] described a net reduction (2e-/2H+) of the C–C π bond at -1.30 V vs. Fc+/0, using a concerted proton-electron transfer (CPET) mediator comprising of cobaltocene ([CpCoII(CpNH)]+) catalyst with a tethered Bronsted base (N,N-dimethylaniline)[65]. This electrochemical approach exhibits high selectivity only in the presence of a synthetically integrated CPET mediator, and two different kinetic mechanistic analyses based on the substrate concentration are revealed: namely rate-limiting CPET followed by an electron-transfer/proton-transfer (ET/PT) step at low concentration and CPET followed by a rate-limiting ET/PT step at high concentration.
Scheme 7. Electrochemical reduction of unsaturated C–C bonds. (A) Electrochemical CPET to C–C bond using a catalytic molecular mediator. Copyright 2021, American Chemical Society[65]; (B) Cobalt-electrocatalytic HAT for hydrogenation of alkenes. Copyright 2022, Springer Nature[66]. CPET: Concerted proton-electron transfer; HAT: hydrogen atom transfer.
In 2022, Gnaim et al. developed a variety of electrochemical [Co–H]-involved hydrogen atom transfer (HAT) protocols using hexafluoroisopropanol (HFIP) as a proton source[66] [Scheme 7B]. This scalable electrochemical sequence enables the selective functionalization of alkenes by adjusting the electrode material, where the metal Mg electrode is more favorable for the reductive hydrogenation of alkenes, and a series of alkenes was then successfully reduced to alkanes in the absence of stoichiometric oxidants. Moreover, large-scale experiments can also be smoothly implemented without strict avoidance of water and oxygen. This electrochemical protocol provides a new perspective on how decades of energy storage research can be utilized to open up electrocatalytic approaches with versatile applications.
Recently, our group presented a new approach for the hydrogenation of aryl alkenes with the three-component system of CH3CN/THF/N,N-diisopropylethylamine (DIEA) at a constant current of 25 mA[67] [Scheme 8A]. This strategy is also tolerant to those substrates (69, 70, 71) containing heteroatom substituents. The detailed mechanism demonstrated the key role of the dual solvent system CH3CN/THF, in which the proportion (v/v = 4:1) of these two solvents is decisive for the alkenes hydrogenation. The DIEA itself is one of the hydrogen sources and also promotes the electrolysis of CH3CN to release hydrogen ions. Afterward, Kolb and Werz developed an operationally simple protocol to realize general site-selective hydrogenation of benzylic alkenes with H2O/D2O as the hydrogen/deuterium source[68] [Scheme 8B]. This method overcomes many limitations (such as the necessity of conjugated EWGs) that possibly appear in classic metal-catalyzed hydrogenation and shows broader substrate applicability (> 50 examples).
Hydrogenation of unactivated alkenes
Compared to the hydrogenation of activated alkenes, the selective electroreduction of unactivated alkenes is more challenging. Unlike the preparation of metal–H by hydride transfer[69], Wu et al. [Scheme 9] reported a unique electroreduction approach to generate [CoIII–H] that allowed a canonical hydrogen evolution reaction while achieving the selective deuteration of alkenes with CD3CO2D as a deuterium source[70]. In addition, the formation process and activity of [CoIII–H] are monitored and studied in detail by systematic electroanalytical [including cyclic voltammetry (CV) and rotating disk electrode (RDE) voltammetry techniques] and spectroscopic investigations [including electron paramagnetic resonance (EPR) and differential electrochemical mass spectrometry (DEMS) experiments]. This work provides a strategy for transition-metal hydrides (M–Hs) as a general platform to participate in a variety of useful electrochemical reactions.
Scheme 9. Electron-initiated hydrogenation of alkenes. Copyright 2022, American Chemical Society[70]. Red numbers: Content of 100% indicates one D atom on one carbon of products.
Hydrogenation of alkynes
The catalytic hydrogenation[71,72] or semihydrogenation[73-75] of alkynes to the corresponding alkenes or alkanes is an extremely important process in the bulk and fine chemical industries. The electroreduction method provides a promising alternative for these metal-facilitated transformations under ambient conditions[76].
Alkynes can also be reduced in some of the alkenes hydrogenation systems described above[61,62,64,66], which will not be repeated here. In 2018, Sherbo et al. disclosed a paired electrolysis of alkynes using a dense palladium membrane, and a reductive deuteration of alkynes was successfully realized with D2O as a deuterium source[77]. The palladium membrane used in this paired electrolysis reaction is impervious to solvent and electrolyte, and two reactions (hydrogenation and electrochemical oxidation reaction) with distinct reaction conditions can be achieved independently in the anode and cathode of a three-compartment cell, respectively. Additionally, this palladium membrane reactor can also be applied to introducing deuterium atoms to afford drug molecules[78] [Scheme 10]. Moreover, no palladium is detected in the reaction mixture determined by inductively coupled plasma-optical emission spectrometry (ICP-OES), even in the amplification experiment, highlighting the great potential of this protocol in drug synthesis.
Scheme 10. Deuterium incorporation and yield by palladium membrane deuteration of alkynes. Copyright 2018, Springer Nature[78]. Red numbers: Content of 100% indicates one D atom on one carbon of products.
In 2019, Li and Ge reported the first example of PdCl2-catalytic semihydrogenation of alkynes to produce a series of Z-alkenes under electrochemical conditions[79]. Moreover, this electroeduction method also enables the hydrogenation of alkynes to produce alkanes when the constant current is increased to 300 mA and the solvent MeOH is replaced by CH3CN. The palladium (0) nanoparticles on the cathode surface and electrochemical reaction solvent are all characterized by scanning electron microscopy (SEM) and X-ray diffraction (XRD) tests and have been demonstrated as the chemisorbed hydrogen carrier.
Interestingly, Wu et al. developed a series of selective electrochemical semihydrogenation (deuteration) systems [Scheme 11] of alkynes (terminal and internal) employing H2O (D2O) as the H (D) source over various Pd-containing alloy cathodes, including Pd-P alloy nanoparticle networks (Pd-P NNs)[80] [Scheme 11A], palladium sulfide nanocapsules (PdSx ANCs)[81] [Scheme 11B and C], and thiolate-modified Pd nanotips (ArS-Pd4S NTs)[82]. The STM image of Pd-P NNs and high-resolution transmission electron microscopy (HRTEM) image of PdSx ANCs are inserted in Scheme 11. These homemade electrodes have a major advantage over the commercially available ones that have appeared in the reported literature on the efficient selective electroreduction of alkynes, in which the doping of the P or S elements in the Pd nanoparticle enhances the specific adsorption of alkynes on the cathode surface and the inherent activity of generating adsorbed atom hydrogen.
Scheme 11. Electrochemical semihydrogenation (deuteration) of various alkynes. (A) Pd-P alloy and H2O are used as the cathode and a hydrogen source. Copyright 2020, Wiley-VCH[80]; (B) Pd-P alloy and D2O are used as the cathode and a hydrogen source. The data in brackets refer to the ratio of yields of the semihydrogenated and full-hydrogenated products; (C) PdSx ANCs and H2O or D2O are used as the cathode and hydrogen sources. Copyright 2022, American Chemical Society[81].
Similarly, Lee et al. realized the semihydrogenation of alkynes with a simple catalyst of earth-abundant nickel [Ni(bpy)3]2+ with excellent Z isomer stereoselectivity under mild conditions[83] [Scheme 12A]. Furthermore, these facile and controllable electrochemical semihydrogenation methods all exhibit strong substrate tolerance, and gram-scale experiments can be performed smoothly, establishing a paradigm for highly efficient electrocatalytic alkynes semihydrogenation in homogeneous or heterogeneous systems. A series of experiments were carried out to study the mechanism, in which (spectro)-electrochemical results indicated that this electrochemical process was promoted by a nickelacyclopropene complex, which was further protonated to give the Ni(II)-vinyl intermediate [Scheme 12B].
Scheme 12. Electrocatalytic semihydrogenation of alkynes with [Ni(bpy)3]2+. (A) Substrate scope; (B) The formation of a nickelacyclopropene complex and Ni(II)-vinyl intermediate. Copyright 2022, American Chemical Society[83].
Other hydrofunctionalization reactions
Hydroboration
As one of the most versatile building blocks, organoboron compounds are widely used in synthetic chemistry[84]. They usually have broad applications, good stability, and rich functional transformations, and thus have won the Nobel Prize in Chemistry twice in 1979 and 2010[85]. Hydroboration of alkenes and alkynes provides a straightforward strategy for synthesizing organoboron compounds[86], and electrochemical hydroboration has attracted increasing attention in recent years[87,88].
Our group developed the first example of aryl alkenes electrochemical hydroboration with pinacolborane (HBpin) with moderate (57%) to excellent (84%) yields[89], in which DIEA-assisted CH3CN for electrolysis and the selection of solvents were the key to the whole hydroboration process [Scheme 13A]. The smooth implementation of the gram-scale experiments and the multi-functional conversions of organoboron compounds further confirm the great potential of this electrochemical method for industrial applications. The detailed deuterium-labeling tests and H2 detection experiments indicate that CH3CN and HBpin are hydrogen sources and the former is the main hydrogen source, which provides a unique strategy for producing hydrogen ions in electrochemical systems. Some key intermediates are captured by a series of radical trapping experiments using 2,2′,6,6′-tetramethyl-1-piperidinyloxy (TEMPO) or the galvinoxyl free radical as the radical scavengers, respectively. Combined with cyclic voltammetric (CV) tests, a possible mechanism of this electrochemical hydroboration is proposed in Scheme 13B. DIEA is first oxidized on the anode surface and then grabs electrons from HBpin and CH3CN, which, in turn, promotes the formation of boron radicals and the release of hydrogen atoms. Subsequently, the boron radical is added to styrene to form a carbon-centered radical intermediate, which again undergoes cathodic reduction and protonation to give the target product.
Scheme 13. Electrochemical hydroboration of aryl alkenes. (A) The scope of substrates; (B) A possible mechanism is proposed. Copyright 2021, Royal Society of Chemistry[89].
Recently, Aelterman et al.[90], Yuan et al.[91], Guo et al.[92] successively reported the electrochemical hydroboration of alkenes with B2Pin2 as a boron source and CH3OH and CH3CN as hydrogen sources, respectively. Unlike HBpin, this diboron reagent exhibits better stability to oxygen and water, which provides more convenience in experimental operation. Under mild conditions or even in the air, versatile borylated building blocks are obtained in good to excellent yields with multiple electrode pairs of different materials, including stainless steel electrodes (SST) cathode and anode, Fe(+)|Al(-), and Fe(+)|Pt(-). However, the electrochemical system using SST as the electrodes is only suitable for activated electron-deficient alkenes. When iron is used as the anode and aluminum or platinum as the cathode, although hydroboration of unactivated alkene can be achieved, two equivalents of KOtBu or one equivalent of CsF is necessary.
For electrochemical boration of alkynes, the products of the only two examples reported so far remain in vinyl boronates, either HBpin or B2pin2 as a hydrogen source. The first electrochemical hydroboration of alkynes was achieved by Aelterman et al. in 2021 using B2Pin2 as the boron source[93] [Scheme 14A]. This cost-effective and catalyst-free method is performed in an undivided cell open to the air. A total of 44 substrates are investigated, showing broad substrate compatibility with excellent yields (yield up to 92%). A putative methoxy-bound boryl radical formed in situ oxidized on the anode surface is involved in the reaction mechanism [Scheme 14B]. Similarly, Qiu et al. also realized the direct hydroboration of alkynes with HBpin as a boron source, carbon as the anode, and platinum as the cathode[94]. The corresponding vinyl boronates can be obtained in good to excellent yields in only 30 min at room temperature.
Scheme 14. Electrochemical hydroboration of alkynes. (A)The scope of substrates; (B) A plausible mechanism is proposed. Copyright 2021, Wiley-VCH[93].
Hydrosilylation
Organosilicon compounds are one of the most valuable precursors and are widely used in modern chemistry, including chemical synthesis, drug development, and polymer production[95,96]. Thus, efficient methods for synthesizing organosilicons are highly desired. The addition of silicon compounds to alkenes and alkynes provides a very profitable process to afford organosilicon[97]. Among them, as a green technology, electrochemistry has also made some progress in the hydrosilylation reaction of unsaturated hydrocarbons[98-100].
In 2020, Lu et al. disclosed the electrochemical synthesis of organosilicon compounds[101], in which the disilylation, silacycle synthesis, hydrosilylation, allylic silylation, and carbonsilylation of alkenes were successfully achieved in the presence of three equivalents of chlorosilane. During the preparation of hydrosilylated products [Scheme 15A], the substrate scope involves conjugated alkenes, alkynes, and chlorosilanes (172-182). The Mg is used as a sacrificial anode to assist the generation of silicon radical intermediates, while CH3CN provides hydrogen protons to complete the alkene hydrosilylation process and the deuterosilylation product could be obtained in the presence of CD3CN. As shown in Scheme 15B, the entire process of incorporating hydrogen atoms and silicon groups into unsaturated C–C bonds is carried out by an electron transfer, chemical protonation, electron transfer, chemical protonation (ECEC) mechanism.
Scheme 15. Electrochemical hydrosilylation of alkenes and alkynes. (A) The scope of substrates; (B) A possible reaction mechanism is proposed. Copyright 2020, American Chemical Society[101].
Using alkynes and Suginome reagent (PhMe2Si–Bpin) as substrates, Biremond et al. realized the electrochemical hydrosilylation of alkynes to prepare vinyl silicates with stainless steel as the anode and cathode[102] [Scheme 16]. This electrochemical method exhibited excellent substrate compatibility and massive unactivated terminal and internal alkynes are smoothly transferred to the corresponding hydrosilylation products. Only the reactivity of internal alkynes was significantly lower than that of terminal alkynes and the yields of the hydrosilylated products of the inner alkynes are only about 50% compared to the high yields (yield up to 91%) of the hydrosilylation reaction of the terminal alkynes. Subsequently, Zhou et al. described an electrochemical hydrosilylation with PhMe2Si–Bpin as a substrate[103], also using the SST material. However, this protocol is limited to the electron-withdrawing alkenes.
Scheme 16. Electrochemical hydrosilylation of alkynes. (A) State of the present work; (B) A possible mechanism is proposed. Copyright 2022, American Chemical Society[102].
Hydroalkylation
Hydroalkylation of alkenes is a new way to build the C–C bond skeleton, allowing the synthesis of molecules with higher molecular complexity, ranging from active molecules to functional polymers[104,105]. Electrochemical synthesis provides a green complementary method to achieve the C–C coupling for the hydroalkylation of alkenes, and significant advances have been received[100].
In 2018, Wu et al. described a TiIII-catalyzed radical alkylation method between electron-deficient alkenes and 2o and 3o alkyl chlorides, in which Lewis acid plays a key role in generating active catalysts[106]. Based on this research result, they used Mg as an anode sacrificial electrode to achieve anti-Markovnikov hydroalkylation reaction of alkenes in the presence of alkyl halide[107] [Scheme 17A]. Such a direct coupling of the Csp3–Csp3 bond with unactivated alkyl halides as substrate is very challenging and rare. The broad substrate range (activated aryl alkenes or 2o and 3o alkyl bromides) confirms the strong compatibility of this electrochemical method for diverse FGs. Similarly, a one-carbon elongation of a terminal alkenes approach with CDCl3 as the substrate was developed by Zhang and Cheng in 2022[108] [Scheme 17B]. Different proton sources give rise to the generation of various alkene hydroalkylation products in moderate to excellent yields.
In 2020, Hu et al. presented a scalable electroreductive alkene-ketone coupling to afford anti-Markovnikov addition product tertiary alcohols with Zn as the anode under environmental conditions[109] [Scheme 18A], in which ketones were added to the unsaturated C–C bond of alkenes as nucleophile reagents. More than 40 examples (alkenes or ketones bearing different substituents) are smoothly investigated and moderated to excellent (yield up to 98%) yields are obtained. Besides, the synthesis of several medicinally relevant structures is also tolerated in this protocol. Interestingly, in a similar type of alkene hydroalkylation addition reaction, Wu et al. realized a substrate-dependent coupling between ketones and alkenes[110] [Scheme 18B]. A series of anti-Markovnikov (linear) and Markovnikov (branched) addition products are successfully obtained in good to excellent yields by adjusting the alkene species and the scale-up experiments at a ten mmol scale under a constant current also confirm the practicability of this method. Additionally, owing to the unique chemical property of benzonitrile derivatives, a range of hydroalkylation products of electron-deficient alkenes are also prepared[111-113].
Hydroalkoxylation
Oxygen-bearing modules are found in a wide range of natural or synthetic bioactive scaffolds[114]. Hydroalkoxylation, namely the addition of alcohols across C–C multiple bonds, is one of the straightforward and efficient routes for synthesizing these building blocks[115-117]. However, compared to other hydrofunctionalization of unsaturated hydrocarbons, the development of electrochemical hydroalkoxylation is relatively slow.
In 2022, Yang et al. developed a cost-effective electrochemical oxidative hydroalkoxylation protocol of alkenes via a Co(II/III/IV) cycle[118] [Scheme 19A]. A large number of hydroalkoxylation products (33 examples including oxygen-hetero or chain) with diverse FGs are obtained in good yields in the absence of stoichiometric chemical oxidants. Mechanical and stereochemical studies demonstrate that an ECEC process is involved in generating CoIV intermediates. Meanwhile, Park et al. described an analogous Co-electrocatalytic hydroalkoxylation approach of alkenes for synthesizing alkyl aryl ethers using phenol as a substrate[119] [Scheme 19B]. The precise control of electrochemical potential and the key role of 1,1,1,3,3,3-hexafluoro-2-propanol (HFIP) as the co-solvent are highlighted in this paper; their combined action promotes the consecutive oxidations of the Co(II) salen catalyst and drives the alkenes to produce carbocationic species. Therefore, the optimal and exclusive chemoselectivity and broad substrate compatibility are smoothly obtained.
Scheme 19. Electrocatalytic oxidative hydroalkoxylation of alkenes. (A) Substrate scope for electrocatalytic hydroalkoxylation of alkenes via Co(II/III/IV) cycle. Copyright 2022, American Chemical Society[118]; (B) Substrate scope for electrocatalytic radical-polar crossover hydroalkoxylation of alkenes with phenols. Copyright 2022, American Chemical Society[119].
Hydrocyanation
Nitriles are key intermediates and precursors in synthesizing valuable compounds, including useful pharmaceuticals and functional materials[120-122]. Alkene hydrocyanation, where H and CN groups are added to the two carbon atoms of the C=C π bond in alkenes, respectively, is an attractive functional transformation to form nitriles. Usually, the selection of toxic hydrocyanation (HCN) as a substrate greatly limits its use in non-industrial laboratories[123]. Song et al. realized a highly efficient Co/Cu dual electrocatalytic enantioselective hydrocyanation of alkenes using trimethylsilyl cyanide (TMSCN) as a nitrile source in 2020[124] [Scheme 20A]. The combination of serine-derived bisoxazolines (sBOXs), Co(salen) complex, and Cu(OTf)2 provides a complementary approach for the synthesis of chiral nitriles [Scheme 20B]. Moreover, sBOXs have been demonstrated to be effective chiral ligands in improving the reaction enantioselectivity and promoting the generation of target chiral nitriles. Under the precise control of the electrochemical voltage, two typical radical reactions (cobalt-mediated hydrogen-atom transfer and copper-promoted radical cyanation) are combined seamlessly at the anode surface, further accomplishing the high enantioselective generation of nitriles with a wide substrate scope. This system opens up a new era for electrochemistry and provides a paradigm for its application in synthesizing high-value fine chemicals.
Scheme 20. Electrochemical enantioselective hydrocyanation of conjugated alkenes. (A) The scope of substrates; (B) A possible mechanism is proposed. Copyright 2020, Springer Nature[124].
Hydrocarboxylation
Direct selective hydrocarboxylation of alkenes and alkynes using carbon dioxide (CO2) as a renewable and non-toxic carbon source is a feasible strategy for synthesizing various high-value-added carboxylic acids[125-127]. So far, transition metal-[128] and photo-catalyzed[129]α- or β-hydrocarboxylation of alkenes has been recognized as an attractive synthetic method for producing carboxylic acids and their derivatives. As a complementary approach, the electrochemical fixation of CO2 across C–C multiple bonds to yield hydrocarboxylation products has gained increasing attention[130-132].
In 2020, Kim et al. realized electrochemical β-selective hydrocarboxylation of styrene using H2O as a clean proton source in the CO2 atmosphere, in which Mg and Ni were used as an anode and cathode in an undivided cell, respectively[133]. In this platform, the addition of H2O significantly improved the β-site selectivity of hydrocarboxylation and inhibited the formation of dicarboxylate products [Scheme 21A]. In terms of selectivity, the ratio of product 227 to 226 increased gradually from 3% to 96% as the H2O concentration increased from 0 to 1 M. However, regarding product yield, the FE of 227 was highest (65%) at 0.1 M H2O and decreased by 47% with 1 M water. The electrochemical β-hydrocarboxylation of other styrene derivatives (0.1 M) was performed in the mixture of CO2-saturated N,N-dimethylformamide (DMF), H2O (0.1 M), and electrolyte nBu4NBF4 (0.1 M) at a 10 mA·cm-2 current density [Scheme 21B].
Scheme 21. Electrochemical β-selective hydrocarboxylation of styrene using CO2 and water. (A) Electrochemical carboxylation of styrene with CO2; (B) The scope of substrates. Copyright 2020, Wiley-VCH[133].
In the same year, Alkayal et al. reported a new and practical electrosynthetic approach for the selective β-hydrocarboxylation [Scheme 22A] of terminal, di- and trisubstituted alkenes[134]. Generally, the electrocarboxylation of styrene with CO2 usually requires a sacrificial anode[133,135], but this electrochemical transformation was conducted on two inert carbon electrodes, with triethanolamine (TEOA) acting as a proton source and sacrificial reducing agent to avoid sacrificing anode. Moreover, a wide variety of styrene derivatives were investigated based on optimized conditions, and good to excellent yields were obtained. In 2022, the same group also achieved electrochemical hydrocarboxylation of α,β-unsaturated esters [Scheme 22B] with high regioselectivity using CO2 as the one-carbon building block[136]. This electrosynthetic platform not only allowed the direct mono-carboxylation of non-aryl α,β-unsaturated esters but also could obtain all-quaternary centered carboxylic acids in good to excellent yields. Notably, the generated products can be purified by simple crystallization without further chromatographic separation. Then, Buckley et al. applied the optimized electrochemical conditions directly to a commercial flow reactor [Scheme 22C], and the target product was obtained at a desired yield (81%), which confirmed the possibility of this method in industrial production.
Scheme 22. Electrochemical hydrocarboxylation of styrene derivatives. (A) Selective β-hydrocarboxylation of substituted olefins. Copyright 2020, American Chemical Society[134]; (B) Selective hydrocarboxylation of α,β-unsaturated esters; (C) Application of the hydrocarboxylation process to the flow synthesis of anti-epilepsy and absence seizure drug ethosuximide precursor 244. Copyright 2021, Wiley-VCH[136].
CONCLUSION AND OUTLOOK
With the development and use of clean and cost-efficient electricity, electrochemical synthesis has been demonstrated as an eco-friendly and energy-renewable tool to achieve various innovative chemical transformations[137-140]. In this review, seven types of viable and economic electrochemical alkene hydrofunctionalization reactions are briefly discussed and summarized. These electrochemical platforms exhibit a particularly broad substrate scope and expansive FG compatibility and avoid the incorporation of stoichiometric redox reagents. To a certain degree, electrosynthesis is recognized as a powerful tool for organic chemistry, and massive functionally valuable chemical reactions with low-cost, high chemo-, regio-, and even stereoselectivities are constructed with high yields smoothly.
However, despite the many advantages of electrochemical reactions, some drawbacks and limitations exist[141]. For example, the involvement of toxic solvents, the necessity of electrolytes, the addition of additives, and the construction of electrochemical devices, to some extent, account for their unfriendliness to industrialization cost and sustainable development. Therefore, based on previous significant advances, further efforts are required to develop more powerful, effective, and harmless electrochemical strategies.
Several promising and potential issues are described in terms of the following: (1) compared to hydrogenation, the development of other hydrofunctionalization strategies from facile alkenes and alkynes has been relatively slower, and more broadly applicable hydrofunctionalization platforms could be explored; (2) enantioselective electrocatalysis for asymmetric synthesis is a new trend, and the development of efficient electrochemical reactions is necessary, which requires the design and preparation of efficient electrochemical chiral catalysts; (3) new electrode materials, reaction mediators, and supporting electrolytes are the key factors to be developed; (4) photoelectrocatalysis provides better group tolerance and controllable redox activity, which will be another boon for the field of synthesis; (5) the design and manufacture of high-throughput electrochemical devices[142] [Scheme 23] will provide the possibility of gram-scale and even industrial preparation of electrochemical products. Beyond these challenges, we believe that various outstanding and innovative results will be achieved in the near future.
Scheme 23. Design strategy of high-throughput electrosynthesis reactor. Copyright 2021, American Chemical Society[142].
DECLARATIONS
Acknowledgments
The authors are grateful for the support from the National Natural Science Foundation of China, DICP Innovation Funding, Dalian Outstanding Young Scientific Talent, and Liaoning Province Doctoral Initiation Fund.
Authors’ contributions
Prepared the manuscript: Zhang Y, Zhao X
Performed manuscript correcting: Qing G
Availability of data and materials
Not applicable.
Financial support and sponsorship
This work was supported by the National Natural Science Foundation of China (21922411 and 22174138), DICP Innovation Funding (DICP-RC201801, I202008, I202243, and I202229), the Dalian Outstanding Young Scientific Talent (2020RJ01), Liaoning Province Doctoral Initiation Fund (2021-BS-009), and the National Key R&D Program of China (Grant No. 2022YFC3400800).
Conflicts of interest
All authors declared that there are no conflicts of interest.
Ethical approval and consent to participate
Not applicable.
Consent for publication
Not applicable.
Copyright
© The Author(s) 2024.
REFERENCES
1. Zhang G, Liu Y, Zhao J, Li Y, Zhang Q. Radical cascade reactions of unsaturated C‒C bonds involving migration. Sci China Chem 2019;62:1476-91.
2. Li G, Huo X, Jiang X, Zhang W. Asymmetric synthesis of allylic compounds via hydrofunctionalisation and difunctionalisation of dienes, allenes, and alkynes. Chem Soc Rev 2020;49:2060-118.
3. Dong B, Shen J, Xie L. Recent developments on 1,2-difunctionalization and hydrofunctionalization of unactivated alkenes and alkynes involving C–S bond formation. Org Chem Front 2023;10:1322-45.
4. Beller M, Seayad J, Tillack A, Jiao H. Catalytic Markovnikov and anti-Markovnikov functionalization of alkenes and alkynes: recent developments and trends. Angew Chem Int Ed Engl 2004;43:3368-98.
5. Enachi A, Baabe D, Zaretzke MK, et al. [(NHC)CoR2]: pre-catalysts for homogeneous olefin and alkyne hydrogenation. Chem Commun 2018;54:13798-801.
6. Sánchez-delgado RA, Rosales M. Kinetic studies as a tool for the elucidation of the mechanisms of metal complex-catalyzed homogeneous hydrogenation reactions. Coordin Chem Rev 2000;196:249-80.
7. Rangheard C, de Julián Fernández C, Phua PH, Hoorn J, Lefort L, de Vries JG. At the frontier between heterogeneous and homogeneous catalysis: hydrogenation of olefins and alkynes with soluble iron nanoparticles. Dalton Trans 2010;39:8464-71.
8. King AO, Larsen RD, Negishi E. Palladium-catalyzed heterogeneous hydrogenation. In: Negishi E, editor. Handbook of organopalladium chemistry for organic synthesis. New York: John Wiley & Sons, Inc.; 2002. pp. 2719-52.
9. Thomas S, Greenhalgh MD. Heterogeneous hydrogenation of C=C and C≡C bonds. In: Knochel P, Molander GA, editors. Comprehensive Organic Synthesis II. Elsevier; 2014. pp. 564-604.
10. Card RJ, Liesner CE, Neckers DC. Poly(styryl)bipyridinepalladium complexes as heterogeneous catalysts for hydrogenation of alkenes and alkynes. J Org Chem 1979;44:1095-8.
11. Lam J, Szkop KM, Mosaferi E, Stephan DW. FLP catalysis: main group hydrogenations of organic unsaturated substrates. Chem Soc Rev 2019;48:3592-612.
12. Li M, Wu W, Jiang H. Recent advances in silver-catalyzed transformations of electronically unbiased alkenes and alkynes. ChemCatChem 2020;12:5034-50.
13. Torres-Calis A, García JJ. Homogeneous manganese-catalyzed hydrofunctionalizations of alkenes and alkynes: catalytic and mechanistic tendencies. ACS Omega 2022;7:37008-38.
14. Chen J, Guo J, Lu Z. Recent advances in hydrometallation of alkenes and alkynes via the first row transition metal catalysis. Chin J Chem 2018;36:1075-109.
15. Pirnot MT, Wang YM, Buchwald SL. Copper hydride catalyzed hydroamination of alkenes and alkynes. Angew Chem Int Ed Engl 2016;55:48-57.
16. Greenhalgh MD, Jones AS, Thomas SP. Iron-catalysed hydrofunctionalisation of alkenes and alkynes. ChemCatChem 2015;7:190-222.
17. Crossley SW, Obradors C, Martinez RM, Shenvi RA. Mn-, Fe-, and Co-catalyzed radical hydrofunctionalizations of olefins. Chem Rev 2016;116:8912-9000.
18. Guo J, Cheng Z, Chen J, Chen X, Lu Z. Iron- and Cobalt-catalyzed asymmetric hydrofunctionalization of alkenes and alkynes. Acc Chem Res 2021;54:2701-16.
19. Yin X, Li S, Guo K, Song L, Wang X. Palladium-catalyzed enantioselective hydrofunctionalization of alkenes: recent advances. Eur J Org Chem 2023;26:e202300783.
20. Müller TE, Hultzsch KC, Yus M, Foubelo F, Tada M. Hydroamination: direct addition of amines to alkenes and alkynes. Chem Rev 2008;108:3795-892.
21. Chiappe C, Capraro D, Conte V, Pieraccini D. Stereoselective halogenations of alkenes and alkynes in ionic liquids. Org Lett 2001;3:1061-3.
22. Choi DS, Kim JH, Shin US, Deshmukh RR, Song CE. Thermodynamically- and kinetically-controlled Friedel-Crafts alkenylation of arenes with alkynes using an acidic fluoroantimonate(v) ionic liquid as catalyst. Chem Commun 2007:3482-4.
23. Balaraman E, Srinivas V, Kumara Swamy K. Hydrophosphonylation of activated alkenes and alkynes via fluoride ion activation in ionic liquid medium. Tetrahedron 2009;65:7603-10.
24. Orella MJ, Román-leshkov Y, Brushett FR. Emerging opportunities for electrochemical processing to enable sustainable chemical manufacturing. Curr Opin Chem Eng 2018;20:159-67.
25. Li G, Lou X, Peng C, Liu C, Chen W. Interface chemistry for sodium metal anodes/batteries: a review. Chem Synth 2022;2:16.
26. Yin Y, Kang X, Han B. Two-dimensional materials: synthesis and applications in the electro-reduction of carbon dioxide. Chem Synth 2022;2:19.
27. Blank S, Nguyen Z, Boucher DG, Minteer SD. Electrochemical cascade reactions for electro-organic synthesis. Curr Opin Electrochem 2022;35:101049.
28. Meyer TH, Choi I, Tian C, Ackermann L. Powering the future: how can electrochemistry make a difference in organic synthesis? Chem 2020;6:2484-96.
29. Wang C, Jiang X, Wang Y, Tang Y, Zhou J, Fu G. Recent advances in nonmetallic modulation of palladium-based electrocatalysts. Chem Synth 2023;3:8.
30. Cheng X, Lei A, Mei T, Xu H, Xu K, Zeng C. Recent applications of homogeneous catalysis in electrochemical organic synthesis. CCS Chem 2022;4:1120-52.
31. Zhong J, Yu Y, Zhang D, Ye K. Merging cobalt catalysis and electrochemistry in organic synthesis. Chinese Chem Lett 2021;32:963-72.
32. Yan M, Kawamata Y, Baran PS. Synthetic organic electrochemical methods since 2000: on the verge of a renaissance. Chem Rev 2017;117:13230-319.
33. Frontana-uribe BA, Little RD, Ibanez JG, Palma A, Vasquez-medrano R. Organic electrosynthesis: a promising green methodology in organic chemistry. Green Chem 2010;12:2099.
34. Blanco DE, Dookhith AZ, Modestino MA. Enhancing selectivity and efficiency in the electrochemical synthesis of adiponitrile. React Chem Eng 2019;4:8-16.
35. Francke R, Little RD. Redox catalysis in organic electrosynthesis: basic principles and recent developments. Chem Soc Rev 2014;43:2492-521.
36. Schiffer ZJ, Manthiram K. Electrification and decarbonization of the chemical industry. Joule 2017;1:10-4.
37. Botte GG. Electrochemical manufacturing in the chemical industry. Electrochem Soc interface 2014;23:49-55.
38. Siu JC, Fu N, Lin S. Catalyzing electrosynthesis: a homogeneous electrocatalytic approach to reaction discovery. Acc Chem Res 2020;53:547-60.
39. Mei H, Yin Z, Liu J, Sun H, Han J. Recent advances on the electrochemical difunctionalization of alkenes/alkynes. Chin J Chem 2019;37:292-301.
40. Novaes LFT, Liu J, Shen Y, Lu L, Meinhardt JM, Lin S. Electrocatalysis as an enabling technology for organic synthesis. Chem Soc Rev 2021;50:7941-8002.
41. Ma C, Fang P, Liu D, et al. Transition metal-catalyzed organic reactions in undivided electrochemical cells. Chem Sci 2021;12:12866-73.
42. Ali T, Wang H, Iqbal W, Bashir T, Shah R, Hu Y. Electro-synthesis of organic compounds with heterogeneous catalysis. Adv Sci 2022;10:e2205077.
43. Jiao KJ, Xing YK, Yang QL, Qiu H, Mei TS. Site-selective C‒H functionalization via synergistic use of electrochemistry and transition metal catalysis. Acc Chem Res 2020;53:300-10.
44. Yuan Y, Lei A. Electrochemical oxidative cross-coupling with hydrogen evolution reactions. Acc Chem Res 2019;52:3309-24.
45. Röckl JL, Pollok D, Franke R, Waldvogel SR. A decade of electrochemical dehydrogenative C,C-coupling of aryls. Acc Chem Res 2020;53:45-61.
46. Yuan Y, Yang J, Lei A. Recent advances in electrochemical oxidative cross-coupling with hydrogen evolution involving radicals. Chem Soc Rev 2021;50:10058-86.
47. Li Y, Wen L, Guo W. A guide to organic electroreduction using sacrificial anodes. Chem Soc Rev 2023;52:1168-88.
48. Xiang H, He J, Qian W, et al. Electroreductively induced radicals for organic synthesis. Molecules 2023;28:857.
49. Yang J, Qin H, Yan K, Cheng X, Wen J. Advances in electrochemical hydrogenation since 2010. Adv Synth Catal 2021;363:5407-16.
50. Shi Z, Li N, Lu H, et al. Recent advances in the electrochemical hydrogenation of unsaturated hydrocarbons. Curr Opin Electrochem 2021;28:100713.
51. Tungler A, Sipos E, Hada V. Heterogeneous catalytic asymmetric hydrogenation of the C=C bond. Curr Org Chem 2006;10:1569-83.
52. Fürstner A. trans-Hydrogenation, gem-hydrogenation, and trans-hydrometalation of alkynes: an interim report on an unorthodox reactivity paradigm. J Am Chem Soc 2019;141:11-24.
54. Lebedeva O, Kultin D, Каlenchuk A, Кustov L. Advances and prospects in electrocatalytic hydrogenation of aromatic hydrocarbons for synthesis of “loaded” liquid organic hydrogen carriers. Curr Opin Electrochem 2023;38:101207.
55. Robertson JC, Coote ML, Bissember AC. Synthetic applications of light, electricity, mechanical force and flow. Nat Rev Chem 2019;3:290-304.
56. Kraft S, Ryan K, Kargbo RB. Recent advances in asymmetric hydrogenation of tetrasubstituted olefins. J Am Chem Soc 2017;139:11630-41.
57. Patil N, Yamamoto Y. Palladium-catalyzed cascade reactions of highly activated olefins. Synlett 2007;2007:1994-2005.
58. Tomida S, Tsuda R, Furukawa S, Saito M, Tajima T. Electroreductive hydrogenation of activated olefins using the concept of site isolation. Electrochem Commun 2016;73:46-9.
59. Huang B, Li Y, Yang C, Xia W. Electrochemical 1,4-reduction of α,β-unsaturated ketones with methanol and ammonium chloride as hydrogen sources. Chem Commun 2019;55:6731-4.
60. Loh YY, Nagao K, Hoover AJ, et al. Photoredox-catalyzed deuteration and tritiation of pharmaceutical compounds. Science 2017;358:1182-7.
61. Liu X, Liu R, Qiu J, Cheng X, Li G. Chemical-reductant-free electrochemical deuteration reaction using deuterium oxide. Angew Chem Int Ed Engl 2020;59:13962-7.
62. Li J, He L, Liu X, Cheng X, Li G. Electrochemical hydrogenation with gaseous ammonia. Angew Chem Int Ed Engl 2019;58:1759-63.
63. Qin Y, Lu J, Zou Z, et al. Metal-free chemoselective hydrogenation of unsaturated carbon–carbon bonds via cathodic reduction. Org Chem Front 2020;7:1817-22.
64. Qin H, Yang J, Yan K, et al. Electrochemical-induced hydrogenation of electron-deficient internal olefins and alkynes with CH3OH as hydrogen donor. Adv Synth Catal 2021;363:2104-9.
65. Derosa J, Garrido-Barros P, Peters JC. Electrocatalytic reduction of C‒C π-bonds via a cobaltocene-derived concerted proton-electron transfer mediator: fumarate hydrogenation as a model study. J Am Chem Soc 2021;143:9303-7.
66. Gnaim S, Bauer A, Zhang HJ, et al. Cobalt-electrocatalytic HAT for functionalization of unsaturated C‒C bonds. Nature 2022;605:687-95.
67. Bi C, Zhao X, Jia Z, et al. Electrochemical reduction of diarylketones and aryl alkenes. ChemCatChem 2023;15:e202300258.
68. Kolb S, Werz DB. Site-selective hydrogenation/deuteration of benzylic olefins enabled by electroreduction using water. Chemistry 2023;29:e202300849.
69. Ananikov VP, Beletskaya IP. Alkyne and alkene insertion into metal–heteroatom and metal–hydrogen bonds: the key stages of hydrofunctionalization process. In: Ananikov V, Tanaka M, editors. Hydrofunctionalization. Berlin: Springer Berlin Heidelberg; 2013. pp. 1-19.
70. Wu X, Gannett CN, Liu J, et al. Intercepting hydrogen evolution with hydrogen-atom transfer: electron-initiated hydrofunctionalization of alkenes. J Am Chem Soc 2022;144:17783-91.
71. Crespo-quesada M, Cárdenas-lizana F, Dessimoz A, Kiwi-minsker L. Modern trends in catalyst and process design for alkyne hydrogenations. ACS Catal 2012;2:1773-86.
72. Moreno-Marrodan C, Liguori F, Barbaro P. Continuous-flow processes for the catalytic partial hydrogenation reaction of alkynes. Beilstein J Org Chem 2017;13:734-54.
73. Michaelides IN, Dixon DJ. Catalytic stereoselective semihydrogenation of alkynes to E-alkenes. Angew Chem Int Ed Engl 2013;52:806-8.
74. Delgado JA, Benkirane O, Claver C, Curulla-Ferré D, Godard C. Advances in the preparation of highly selective nanocatalysts for the semi-hydrogenation of alkynes using colloidal approaches. Dalton Trans 2017;46:12381-403.
75. Liu Z, Zhang L, Ren Z, Zhang J. Advances in selective electrocatalytic hydrogenation of alkynes to alkenes. Chemistry 2023;29:e202202979.
76. Bortolami M, Petrucci R, Rocco D, Scarano V, Chiarotto I. Alkynes as building blocks, intermediates and products in the electrochemical procedures since 2000. ChemElectroChem 2021;8:3604-13.
77. Sherbo RS, Delima RS, Chiykowski VA, Macleod BP, Berlinguette CP. Complete electron economy by pairing electrolysis with hydrogenation. Nat Catal 2018;1:501-7.
78. Kurimoto A, Sherbo RS, Cao Y, Loo NWX, Berlinguette CP. Electrolytic deuteration of unsaturated bonds without using D2. Nat Catal 2020;3:719-26.
79. Li B, Ge H. Highly selective electrochemical hydrogenation of alkynes: rapid construction of mechanochromic materials. Sci Adv 2019;5:eaaw2774.
80. Wu Y, Liu C, Wang C, Lu S, Zhang B. Selective transfer semihydrogenation of alkynes with H2O (D2O) as the H (D) source over a Pd-P cathode. Angew Chem Int Ed Engl 2020;59:21170-5.
81. Li H, Gao Y, Wu Y, et al. σ-Alkynyl adsorption enables electrocatalytic semihydrogenation of terminal alkynes with easy-reducible/passivated groups over amorphous PdSx nanocapsules. J Am Chem Soc 2022;144:19456-65.
82. Gao Y, Yang R, Wang C, et al. Field-induced reagent concentration and sulfur adsorption enable efficient electrocatalytic semihydrogenation of alkynes. Sci Adv 2022;8:eabm9477.
83. Lee MY, Kahl C, Kaeffer N, Leitner W. Electrocatalytic semihydrogenation of alkynes with [Ni(bpy)3]2+. JACS Au 2022;2:573-8.
84. Bage AD, Nicholson K, Hunt TA, Langer T, Thomas SP. The hidden role of boranes and borohydrides in hydroboration catalysis. ACS Catal 2020;10:13479-86.
85. Bose SK, Mao L, Kuehn L, et al. First-row d-block element-catalyzed carbon‒boron bond formation and related processes. Chem Rev 2021;121:13238-341.
86. Zuo Z, Wen H, Liu G, Huang Z. Cobalt-catalyzed hydroboration and borylation of alkenes and alkynes. Synlett 2018;29:1421-9.
87. Moniruzzaman M, Afrin S, Ali MK. Progress in the electrochemical synthesis of organoboron compounds. Asian J Org Chem 2023;12:e202300090.
88. Yin C, Tang S, Mei J, Hu X, Zhang H. Electrochemical synthesis and transformation of organoboron compounds. Org Chem Front 2023;10:3361-77.
89. Zhang Y, Zhao X, Bi C, et al. Selective electrocatalytic hydroboration of aryl alkenes. Green Chem 2021;23:1691-9.
90. Aelterman M, Jubault P, Poisson T. Electrochemical borylation of electron-deficient alkenes and allenoates. Eur J Org Chem 2023;26:e202300063.
91. Yuan Q, Zhang X, Guo L, Yang C, Xia W. Metal-free electrochemical hydroboration of olefins. Adv Synth Catal 2023;365:1788-93.
92. Guo C, Li P, Wang S, et al. Selective electroreductive hydroboration of olefins with B2pin2. J Org Chem 2023;88:4569-80.
93. Aelterman M, Sayes M, Jubault P, Poisson T. Electrochemical hydroboration of alkynes. Chemistry 2021;27:8277-82.
94. Qiu H, Lv K, Qu H, et al. Chemoselective electrocatalytic hydroboration of alkynes with pinacolborane. J Mol Struct 2022;1266:133463.
95. Pal PP, Ghosh S, Hajra A. Recent advances in carbosilylation of alkenes and alkynes. Org Biomol Chem 2023;21:2272-94.
96. Li L, Huang W, Xu Z, Xu L. Catalytic asymmetric silicon‒carbon bond-forming transformations based on Si‒H functionalization. Sci China Chem 2023;66:1654-87.
97. Sun J, Deng L. Cobalt complex-catalyzed hydrosilylation of alkenes and alkynes. ACS Catal 2016;6:290-300.
98. Jouikov V, Grigorieva L. Electrochemically induced silylation of unsaturated compounds. Electrochimica Acta 1996;41:469-70.
99. Kuciński K. Electrifying synthesis of organosilicon compounds - from electrosynthesis to electrocatalysis. Inorg Chem Front 2023;10:1382-94.
100. Zhang W, Guan W, Martinez Alvarado JI, Novaes LFT, Lin S. Deep electroreductive chemistry: harnessing carbon- and silicon-based reactive intermediates in organic synthesis. ACS Catal 2023;13:8038-48.
101. Lu L, Siu JC, Lai Y, Lin S. An electroreductive approach to radical silylation via the activation of strong Si‒Cl bond. J Am Chem Soc 2020;142:21272-8.
102. Biremond T, Jubault P, Poisson T. Electrochemical hydrosilylation of alkynes. ACS Org Inorg Au 2022;2:148-52.
103. Zhou H, Fei L, Zhang J, Pan Y, Tang H. Electrochemical hydrosilylation of electron-withdrawing alkenes. Adv Synth Catal 2023;365:1591-5.
104. Yang KS, Gurak JA Jr, Liu Z, Engle KM. Catalytic, regioselective hydrocarbofunctionalization of unactivated alkenes with diverse C‒H nucleophiles. J Am Chem Soc 2016;138:14705-12.
105. Yang D, Huang H, Li MH, et al. Directed cobalt-catalyzed anti-markovnikov hydroalkylation of unactivated alkenes enabled by “Co‒H” catalysis. Org Lett 2020;22:4333-8.
106. Wu X, Hao W, Ye KY, et al. Ti-catalyzed radical alkylation of secondary and tertiary alkyl chlorides using michael acceptors. J Am Chem Soc 2018;140:14836-43.
107. Zhang W, Lin S. Electroreductive carbofunctionalization of alkenes with alkyl bromides via a radical-polar crossover mechanism. J Am Chem Soc 2020;142:20661-70.
108. Zhang X, Cheng X. Electrochemical reductive functionalization of alkenes with deuterochloroform as a one-carbon deuteration Block. Org Lett 2022;24:8645-50.
109. Hu P, Peters BK, Malapit CA, et al. Electroreductive olefin‒ketone coupling. J Am Chem Soc 2020;142:20979-86.
110. Wu H, Chen W, Deng W, et al. Cathodic regioselective coupling of unactivated aliphatic ketones with alkenes. Org Lett 2022;24:1412-7.
111. Xu H, Liu J, Nie F, Zhao X, Jiang Z. Metal-free hydropyridylation of thioester-activated alkenes via electroreductive radical coupling. J Org Chem 2021;86:16204-12.
112. Zhang S, Gao W, Shi J, et al. Regioselective umpolung addition of dicyanobenzene to α,β-unsaturated alkenes enabled by electrochemical reduction. Org Chem Front 2022;9:1261-6.
113. Zhang S, Li L, Li X, et al. Electroreductive 4-pyridylation of electron-deficient alkenes with assistance of Ni(acac)2. Org Lett 2020;22:3570-5.
114. Nanda SK, Mallik R. Transition metal-catalyzed hydroalkoxylation of alkynes: an overview. Chemistry 2021;27:15571-604.
115. Goodwin JA, Aponick A. Correction: Regioselectivity in the Au-catalyzed hydration and hydroalkoxylation of alkynes. Chem Commun 2016;521:6731.
116. Xie W, Li Z. Asymmetric synthesis of ethers by catalytic alkene hydroalkoxylation. Synthesis 2020;52:2127-46.
117. Kennemur JL, Maji R, Scharf MJ, List B. Catalytic asymmetric hydroalkoxylation of C‒C multiple bonds. Chem Rev 2021;121:14649-81.
118. Yang F, Nie Y, Liu H, Zhang L, Mo F, Zhu R. Electrocatalytic oxidative hydrofunctionalization reactions of alkenes via Co(II/III/IV) cycle. ACS Catal 2022;12:2132-7.
119. Park SH, Jang J, Shin K, Kim H. Electrocatalytic radical-polar crossover hydroetherification of alkenes with phenols. ACS Catal 2022;12:10572-80.
120. Fleming FF, Yao L, Ravikumar PC, Funk L, Shook BC. Nitrile-containing pharmaceuticals: efficacious roles of the nitrile pharmacophore. J Med Chem 2010;53:7902-17.
121. Wang T, Jiao N. Direct approaches to nitriles via highly efficient nitrogenation strategy through C‒H or C‒C bond cleavage. Acc Chem Res 2014;47:1137-45.
122. Wang MX. Enantioselective biotransformations of nitriles in organic synthesis. Acc Chem Res 2015;48:602-11.
123. Strache JP, Münzer L, Adler A, Blunk D, Schmalz H. Enantioselective nickel-catalyzed hydrocyanation of homostilbenes. Eur J Org Chem 2023;26:e202300050.
124. Song L, Fu N, Ernst BG, et al. Dual electrocatalysis enables enantioselective hydrocyanation of conjugated alkenes. Nat Chem 2020;12:747-54.
125. Zhang Y, Riduan SN. Catalytic hydrocarboxylation of alkenes and alkynes with CO2. Angew Chem Int Ed Engl 2011;50:6210-2.
126. Xiao W, Zhang J, Wu J. Recent advances in reactions involving carbon dioxide radical anion. ACS Catal 2023;13:15991-6011.
127. Xu QJ, Jiang JW, Wang XF, Duan LY, Guo H. Understanding oxygen vacant hollow structure CeO2@In2O3 heterojunction to promote CO2 reduction. Rare Met 2023;42:1888-98.
128. Gaydou M, Moragas T, Juliá-Hernández F, Martin R. Site-selective catalytic carboxylation of unsaturated hydrocarbons with CO2 and water. J Am Chem Soc 2017;139:12161-4.
129. Seo H, Liu A, Jamison TF. Direct β-selective hydrocarboxylation of styrenes with CO2 enabled by continuous flow photoredox catalysis. J Am Chem Soc 2017;139:13969-72.
130. Wang S, Feng T, Wang Y, Qiu Y. Recent advances in electrocarboxylation with CO2. Chem Asian J 2022;17:e202200543.
131. Liu X, Zhang K, Tao L, Lu X, Zhang W. Recent advances in electrochemical carboxylation reactions using carbon dioxide. Green Chem Eng 2022;3:125-37.
132. Senboku H. Electrochemical fixation of carbon dioxide: synthesis of carboxylic acids. Chem Rec 2021;21:2354-74.
133. Kim Y, Park GD, Balamurugan M, Seo J, Min BK, Nam KT. Electrochemical β-selective hydrocarboxylation of styrene using CO2 and water. Adv Sci 2020;7:1900137.
134. Alkayal A, Tabas V, Montanaro S, Wright IA, Malkov AV, Buckley BR. Harnessing applied potential: selective β-hydrocarboxylation of substituted olefins. J Am Chem Soc 2020;142:1780-5.
135. Wang H, Lin M, Fang H, Chen T, Lu J. Electrochemical dicarboxylation of styrene: synthesis of 2-phenylsuccinic acid. Chin J Chem 2007;25:913-6.
136. Sheta AM, Alkayal A, Mashaly MA, et al. Selective electrosynthetic hydrocarboxylation of α,β-unsaturated esters with carbon dioxide**. Angew Chem Int Ed Engl 2021;60:21832-7.
137. Liu J, Xiao HZ, Fu Q, Yu DG. Advances in radical phosphorylation from 2016 to 2021. Chem Synth 2021;1:9.
138. Zhong G, Huang Y, He L. Regioselectivity of N-heteroarene electrocarboxylations: divided vs. undivided cell. Chem Synth 2023;3:19.
139. Liu W, Niu X, Tang J, et al. Energy-efficient anodic reactions for sustainable hydrogen production via water electrolysis. Chem Synth 2023;3:44.
140. Li S, Du Y, Wang M, et al. Optimizing the reaction pathway of nitride electrode by co-doping strategy for boosting alkaline hydrogen evolution reaction kinetics. Sci China Mater 2023;66:4639-49.
141. Yuan Y, Lei A. Is electrosynthesis always green and advantageous compared to traditional methods? Nat Commun 2020;11:802.
Cite This Article
Export citation file: BibTeX | RIS
OAE Style
Zhang Y, Zhao X, Qing G. Electrochemical-induced hydrofunctionalizations of alkenes and alkynes. Chem Synth 2024;4:17. http://dx.doi.org/10.20517/cs.2023.38
AMA Style
Zhang Y, Zhao X, Qing G. Electrochemical-induced hydrofunctionalizations of alkenes and alkynes. Chemical Synthesis. 2024; 4(2): 17. http://dx.doi.org/10.20517/cs.2023.38
Chicago/Turabian Style
Zhang, Yahui, Xiangyu Zhao, Guangyan Qing. 2024. "Electrochemical-induced hydrofunctionalizations of alkenes and alkynes" Chemical Synthesis. 4, no.2: 17. http://dx.doi.org/10.20517/cs.2023.38
ACS Style
Zhang, Y.; Zhao X.; Qing G. Electrochemical-induced hydrofunctionalizations of alkenes and alkynes. Chem. Synth. 2024, 4, 17. http://dx.doi.org/10.20517/cs.2023.38
About This Article
Copyright
Author Biographies
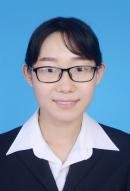
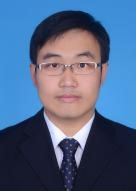
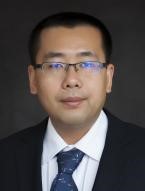
Data & Comments
Data
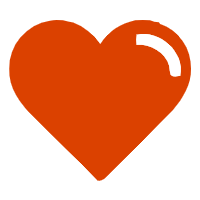

Comments
Comments must be written in English. Spam, offensive content, impersonation, and private information will not be permitted. If any comment is reported and identified as inappropriate content by OAE staff, the comment will be removed without notice. If you have any queries or need any help, please contact us at support@oaepublish.com.