Constructing a hierarchical MoS2/MXene heterostructure for efficient capacitive deionization of saline water
Abstract
Well-dispersed and few-layered molybdenum disulfide (MoS2) has been considered as a suitable electrode material for pseudocapacitive capacitive deionization (CDI) due to its lamellar structure, flexible interlayer intercalation, and high theoretical capacity. However, the serious aggregation, low electrical conductivity and poor hydrophilicity of MoS2 have limited its desalination application. To address these issues, few-layered MXene is used as a conductive and hydrophilic skeleton to support MoS2 nanosheets. In this study, a hierarchical MoS2/MXene heterostructure is fabricated for highly efficient CDI of saline water. The designed heterostructure effectively inhibits the agglomeration of MoS2 and MXene nanosheets, exposes more active electrochemical sites, and improves the wettability, thereby enhancing ion/charge transport. The MoS2/MXene heterostructure electrode exhibits low electrical resistance (3.2 Ω), along with a high specific capacitance of 171.8 F·g-1 at 2 A·g-1. Furthermore, the MoS2/MXene-based CDI electrode demonstrates an excellent desalination capacity of
Keywords
INTRODUCTION
In recent years, the indiscriminate discharge of industrial wastewater, the misuse of various chemical fertilizers and pesticides, and other harmful substances to the environment have led to an increasing pollution of water sources, resulting in a scarcity of freshwater resources. Exploring highly efficient seawater/brackish desalination techniques is a crucial strategy to address the freshwater shortage. However, traditional desalination techniques, such as pressure-driven desalination, thermal-driven desalination and electric-driven desalination, are hindered by the challenges including high cost, excessive energy consumption, and issues related to secondary pollution. These limitations make them unsuitable for practical applications[1-3]. Capacitive deionization (CDI), as a novel and emerging desalination technique, is characterized by easy operation, cost-effectiveness, minimal energy consumption, and eco-friendly features, making it highly promising for seawater desalination and heavy metal removal[4,5].
The CDI process is typically categorized into two main stages: adsorption and desorption[6]. During the adsorption stage, a positive voltage is applied to CDI. Cations and anions in saltwater move towards negative and positive electrodes, respectively, due to the applied electrostatic field. These results in the ions being adsorbed onto the surface or pores of electrode materials. In the desorption stage, the ions adsorbed on electrode materials are released back into water when the charge is removed or reversed[7]. The key CDI components are the electrode materials, which can be categorized into double-layer capacitance materials (e.g., graphene, carbon nanotubes, and carbon aerogels)[5,8-11] and faradic electrode materials (e.g., transition metal oxides/sulfides and magnesium titanate)[12-15]. The double-layer capacitance materials primarily rely on ion storage to remove salts from water, leading to insufficient desalination capacity, non-selective adsorption of anions and cations, and anode corrosion[16]. In addition, co-ion repulsion effects and side reactions in these materials result in relatively low charge utilization efficiency. To address these limitations, novel faradic electrode materials, such as transition metal oxides, sulfides, carbides, redox-active polymers, and Prussian blue, have been prepared to improve the inherent desalination performances[17-22].
MXene is a novel family of 2D materials composed of transition-metal layers interleaved with carbide/nitride ones[23]. It is commonly prepared by selectively removing the “A” layer from the MAX phase through etching. In the general formula of Mn+1AXn
Molybdenum disulfide (MoS2) is a typical 2D layered material composed of two hexagonal nanosheets (S atoms) and one intermediate hexagonal sheet (Mo atoms). The weak van der Waals force between neighboring layers of MoS2 can enhance ion adsorption and transport. MoS2-based electrodes have shown excellent CDI performance in capturing sodium ions from salt water[12,32-37]. However, the serious structural aggregation, inferior electrical conductivity, and poor hydrophilicity of MoS2 greatly limit its desalination performance.
In this work, few-layered MXene is utilized as a robust, conductive and hydrophilic skeleton to support MoS2 nanosheets for the fabrication of hierarchical MoS2/MXene heterostructures. The designed MoS2/MXene heterostructures can prevent the aggregation of both MXene and MoS2 nanosheets and provide a rich exposure of active sites, expanded MXene interlayer spacing, and suitable wettability, enabling fast ion diffusion. As a result, the optimized MoS2/MXene device for CDI exhibits an excellent desalination capacity of 55.8 mg·g-1 at a voltage of 1.2 V. Therefore, high conductivity and hydrophilicity MXene-supported nanosized MoS2 shows great promise as a low-cost electrode material in the field of CDI.
EXPERIMENTAL SECTION
Preparation of MXene nanosheets
MXene nanosheets are prepared by selectively eliminating the Al layer from Ti3AlC2 in an HF aqueous solution, following the method reported in our prior work[38]. The specific procedures can be found in the Supplementary Materials.
Synthesis of MoS2/MXene heterostructures
The hierarchical MoS2/MXene heterostructures are synthesized using a straightforward solvothermal reaction method. First of all, MXene nanosheets are gradually dispersed into deionized water using ultrasonic dispersion under Ar atmosphere. Then, 0.22 g of (NH4)6Mo7O24·4H2O is added into the MXene nanosheet dispersion via 0.5 h ultrasonic dispersion for in situ anchoring Mo ions. Afterward, 0.76 g of thiourea is incorporated into the above dispersion and homogeneously mixed by 0.5 h magnetic stirring under an Ar atmosphere. The dispersion obtained is transferred into a 50 mL Teflon stainless-steel autoclave and reacted at 200 °C for 12 h. The hierarchical MoS2/MXene heterostructure is achieved through centrifugation, followed by multiple washes and vacuum drying. The resulting hierarchical MoS2/MXene heterostructures with MoS2 and MXene weight ratios of 1:1, 1:2, and 1:4 are labeled as MoS2/MXene-1, MoS2/MXene-2, and MoS2/MXene-4, respectively. For comparison, pure MoS2 nanosheets are prepared under the same conditions but without MXene nanosheets.
Characterization
The detailed characterizations are described in the Supplementary Materials.
Electrochemical measurements and desalination measurements
The details of electrochemical measurements and desalination measurements are described in the Supplementary Materials.
RESULTS AND DISCUSSIONS
Preparation, morphologies, and structures of MoS2/MXene heterostructures
As shown in Figure 1, Ti3C2Tx MXene is produced by selectively etching the Al layer in Ti3AlC2 using HF as the etchant. The surface of MXene nanosheets is rich in -F, -OH, and/or -O groups, which can enhance the hydrophilicity performance and provide abundant growth sites for MoS2 nanosheets. Thus, 2D MXene and MoS2 nanosheets can form a mutually reinforcing structure to prevent aggregation of the nanosheets. MXene nanosheets act as a robust, hydrophilic and conductive substrate, while MoS2 ones act as pillars between MXene nanosheets, effectively preventing MXene self-accumulation and providing a pathway for rapid ion transport. Therefore, the obtained hierarchical MoS2/MXene heterostructure shows great promise as a promising CDI electrode material.
Figure 1. Schematic for the preparation of hierarchical MoS2/MXene heterostructure. MoS2: Molybdenum disulfide.
The synthesized MXene nanosheets exhibit an accordion-like structure [Supplementary Figure 1A] after selective etching the Al layer in Ti3AlC2 with HF solution, confirming the successful preparation of multi-layered MXene nanosheets. Pure MoS2 consists of large-sized nanosheets that are randomly stacked and aggregated into a typical flower-like nanostructure [Supplementary Figure 1B]. However, the hierarchical MoS2/MXene heterostructures with varying amounts of MoS2 loading exhibit different morphologies [Figure 2]. Following a one-step hydrothermal reaction of MXene nanosheets in Mo salt solution, small MoS2 nanosheets are uniformly inserted into the interlayers of 2D conductive MXene nanosheets, forming mutually supported architectures. The MoS2 nanosheets play a certain role in supporting MXene nanosheets and preventing their restacking. Meanwhile, the anchoring sites provided by MXene nanosheets also restrict the self-stacking of the MoS2 nanosheets. Thus, mutually supported architectures formed by MXene nanosheets and MoS2 nanosheets can offer abundant active sites for ion adsorption. With an increased dosage of Mo salt, more MoS2 nanosheets form on the surface and interlayer of MXene nanosheets. Notably, when the weight ratio is moderate, MoS2 nanosheets are uniformly grown on MXene substrate
Figure 2. SEM images of (A and B) MoS2/MXene-1, (C and D) MoS2/MXene-2, (E and F) MoS2/MXene-4 with low and high magnifications, and EDX mapping of MoS2/MXene-2. SEM: Scanning electron microscope; MoS2: molybdenum disulfide; EDX: energy-dispersive X-ray spectroscopy.
Furthermore, energy-dispersive X-ray spectroscopy (EDX) mapping of the hierarchical MoS2/MXene heterostructure [Figure 2G-L] confirms the presence and uniform distribution of Ti, C, Mo, S, and O elements. The MoS2 nanosheets are uniformly distributed on the surface and interlayer of MXene nanosheets. A transmission electron microscopy (TEM) image clearly shows the typical layered structures of the heterostructure [Supplementary Figure 2A]. Additionally, a high-resolution TEM (HRTEM) image
Figure 3 illustrates the X-ray diffraction (XRD) patterns of Ti3AlC2, MXene, MoS2 and MoS2/MXene. In the XRD pattern of Ti3AlC2, the peaks at 9.5°, 19.1°, 34.0°, 39.0°, 41.8°, and 60.8° correspond to (002), (004), (101), (104), (105), and (110) planes of Ti3AlC2, respectively. However, the peak at 39.0° disappears after HF etching, indicating the formation of Ti3C2Tx. Importantly, the diffraction peaks shift from 9.5° and 19.1° to 8.9° and 18.2°, respectively. According to the Bragg equation, the interlayer space of MXene nanosheets [(002) plane] increases from 18.6 to 19.7 Å compared with Ti3AlC2 [Figure 3B]. The expanded interlayer space of MXene can provide large space for anchoring MoS2 nanosheets. As shown in Figure 3A, the MoS2/MXene heterostructures exhibit diffraction peaks corresponding to the (002), (004), (006), (008) and (110) planes of MXene, and prominent peaks at 14°, 33.8°, and 59.3°, which correspond to (002), (100), and (110) planes of MoS2 nanosheets, respectively. Thus, the hierarchical MoS2/MXene heterostructures show characterization diffraction peaks of both MXene and MoS2 nanosheets. Furthermore, XRD patterns reveal a distinct diffraction peak at 25.4°, attributing to the (101) plane of TiO2, indicating that a portion of MXene has undergone oxidation to form TiO2 during the hydrothermal process. After the intercalation of MoS2 nanosheets, the (002) plane diffraction peak of MXene further shifts, resulting in an increase in layer spacing from 18.6 Å to 24.6-24.7 Å compared to Ti3AlC2. The enlarged interlayer spacing is attributed to the exfoliation effect of MoS2 nanosheets. The larger layer spacing can provide a larger ion channel, which can facilitate the rapid ion diffusion and improve the ion adsorption rate in the desalination process.
Figure 3. XRD patterns of Ti3AlC2, MXene nanosheets, MoS2 nanosheets, and hierarchical MoS2/MXene heterostructures. XRD: X-ray diffraction; MoS2: molybdenum disulfide.
In Figure 4A, the survey scan reveals the existence of C, O, Mo, S, and Ti elements in MoS2/MXene. The C spectrum [Figure 4B] shows four main peaks at 288.7, 286.2, 284.5, and 283.2 eV, which are ascribed to
Electrochemical performances of MoS2/MXene heterostructures
Figure 5A displays cyclic voltammetry (CV) curves of MXene and MoS2/MXene at a scan rate of 20 mV·s-1 in the potential range of -0.8 to 0.4 V. The CV curve of MXene shows no distinct redox peak and has a distorted rectangular shape, indicating the contribution of electrical double-layer capacitance in MXene electrode. However, the CV curves of MoS2/MXene with different MoS2 nanosheet loadings exhibit minor redox peaks between -0.3 and -0.1 V, indicating the pseudocapacitance behavior of MoS2 nanosheets. The region enclosed by these curves within the potential range represents the relative specific capacitance. As shown in Figure 5A, the areas under the CV curves of MoS2/MXene are larger than that of MXene. Among them, MoS2/MXene-2 has the largest area, suggesting the highest specific capacitance. The superior electrochemical performance of MoS2/MXene-2 can be attributed to the enhanced hydrophilicity and conductivity and offered by MXene, along with the optimized amount of MoS2 nanosheets. The CV curves of MXene and MoS2/MXene heterostructures at different scan rates are shown in Figure 5B-E. All these curves present rectangular mirror images, indicating excellent electrochemical behavior at diverse scan rates. Moreover, a noticeable increase in current density was observed with higher scan rates. Analysis of the CV curves indicates that MoS2/MXene-2 also has the best specific capacitances among the tested materials at the respective scan rates [Figure 5F]. The improved specific capacitance can be ascribed to multiple factors. Firstly, the interconnected framework formed by the combination of MoS2 and MXene nanosheets promotes charge transfer in the hierarchical MoS2/MXene heterostructure. Additionally, the in situ anchoring effect of MXene provides growth sites for MoS2 nanosheets, effectively inhibiting the self-aggregation of MoS2 and MXene nanosheets, while also offering more exposed redox sites.
Figure 5. (A) CV curves of MXene and MoS2/MXene heterostructure electrodes at a scan rate of 20 mV·s-1; (B) CV curves of MXene, (C) MoS2/MXene-1, (D) MoS2/MXene-2, (E) MoS2/MXene-4 at various scan rates; (F) the specific capacitances calculated from CV curves. CV: Cyclic voltammetry; MoS2: molybdenum disulfide.
The galvanostatic charge-discharge (GCD) curves at a current density of 2 A·g-1 are shown in Figure 6A. The specific capacitances of MXene, MoS2/MXene-1, MoS2/MXene-2, and MoS2/MXene-4 electrodes calculated from these curves are 37.8, 50.3, 171.8, and 124.7 F·g-1, respectively. The trend in the GCD performances agrees with the findings from the CV curves. Figure 6B-E exhibits GCD curves at varying current densities, and Figure 6F summarizes the specific capacitances calculated from these GCD curves. Clearly, the MoS2/MXene-2 electrode shows the best electrochemical performance. For comparison, a pure MoS2 electrode was also examined as a reference measuring by CV and GCD curves [Supplementary Figure 3]. The CV curves of MoS2 indicate the presence of pseudocapacitance in MoS2. The specific capacitance of MoS2, determined from the CV curves, rises with increasing scan rates. Similarly, the specific capacitance of MoS2, derived from the GCD curves
Figure 6. (A) Charge/discharge curves of MXene and hierarchical MoS2/MXene heterostructure electrodes at a current density of
Electrochemical impedance spectroscopy (EIS) was further explored to analyze the conductive performance and electron/ion transport behaviors at the electrodes/electrolyte interface. As seen in [Supplementary Figure 4], all Nyquist plots exhibit subtle arcs in high-frequency regions and linear trends in low-frequency regions. In low-frequency regions, a steeper line indicates faster ion diffusion within the electrode. The high-frequency arc is associated with electronic resistance, while the subtle arc likely results from charge transfer resistance. MoS2/MXene-2 electrode shows the lowest surface resistance (3.2 Ω), while the MXene, MoS2/MXene-1, MoS2/MXene-2 and MoS2/MXene-4 ones exhibit resistance values of 9.4, 4.9, and 4.7 Ω, respectively. The charge transfer resistance (Rct) of MoS2/MXene-2 electrode shows 4.4 Ω by fitting the equivalent circuit, while MXene, MoS2/MXene-1, and MoS2/MXene-4 exhibit resistance values of 10.3, 5.9, and 5.5 Ω, respectively. This demonstrates that the incorporation of MoS2 nanosheets into the MXene can reduce both the surface resistance and charge transfer resistance. The presence of MoS2 nanosheets acts as pillars, effectively inhibiting the aggregation of MXene and expanding its interlayer distance. Therefore, MoS2/MXene heterostructure electrodes show lower resistance compared to pure MXene electrodes.
CDI performances of MoS2/MXene heterostructures
Surface wettability is essential for the penetration of electrolytes into electrode materials, thus influencing CDI performance. As seen in [Supplementary Figure 5], MXene nanosheets exhibit hydrophilic (water contact angle = 26.5°) as a result of the abundance of oxygenic groups and fluorine groups from their surface. On the other hand, MoS2 nanosheets are hydrophobic, with a water contact angle of 112.1°. The introduction of hydrophilic MXene significantly reduces the water contact angle of the hierarchical MoS2/MXene heterostructure. The improved surface wettability can enhance the utilization of the active sites and further increase the desalination capacity and desalination rate.
To assess the desalination performances, desalination experiments were conducted in batch mode using different voltages/concentrations in NaCl solution. Prior to evaluating the desalination performance, the changes in NaCl solution conductivity at varied concentrations were initially studied. A conductivity meter was used to test the conductivity of NaCl solutions with specified concentrations. Subsequently, the relationship between the concentration of NaCl solution and their conductivity at room temperature was established [Supplementary Figure 6]. As shown in Figure 7A-E and Supplementary Figure 7, the desalination capacities increase significantly with the applied voltage. Moreover, higher desalination capacities are generated at higher conductivities of the NaCl solution. Several factors contribute to the enhanced desalination performance. Primarily, the high voltage can not only facilitate the ion transport and diffusion into the inner layers of MXene and MoS2 nanosheets but also increase the active sites for ion adsorption. The high conductivity of NaCl solution means not only high NaCl concentration but also more ions and a large concentration gradient. Thus, all these factors facilitate the enhancement of desalination capacity. As shown in Figure 7E, the desalination capacities of hierarchical MoS2/MXene heterostructure devices are much higher than those of MXene devices and MoS2 devices [Supplementary Figure 7B], and the MoS2/MXene-2 device exhibits the highest desalination capacity (up to 55.8 mg·g-1 at a voltage of 1.2 V and an initial conductivity of 1,000 μS·cm-1). These results suggest that the MoS2/MXene-2 device exhibits the optimal candidate for CDI.
Figure 7. The desalination capacity and time curves of (A) MXene, (B) MoS2/MXene-1, (C) MoS2/MXene-2, (D) MoS2/MXene-4 devices in NaCl solution; (E) The desalination capacities and (F) the CDI working process of MXene and hierarchical MoS2/MXene heterostructure devices. MoS2: Molybdenum disulfide.
Figure 7F shows the CDI behavior of hierarchical MoS2/MXene heterostructure anode and cathode. In the CDI desalination process, Na+ ions are intercalated into the MXene and MoS2 nanosheet interlay, while Cl- ions are intercalated into the active carbon. As mentioned in the previous analysis discussion, MoS2/MXene-2 exhibits an optimized hierarchical structure characterized by even distribution of MoS2 nanosheets on MXene, along with increased exposed active edges. The highly hydrophilic MXene serves as a conductive substrate, which can reduce the ion diffusion paths. Besides, the pillar effect of MoS2 can greatly inhibit the restacking of MXene and enlarge its interlayer distance. Furthermore, the in situ anchoring effect of MXene guides the growth of MoS2 nanosheets, effectively preventing their restacking. For MoS2/MXene-4, only a few MoS2 nanosheets are dispersed on the surface and between the layers of MXene, resulting in a reduced number of active sites for desalination. Thus, the desalination capacity of MoS2/MXene-4 devices is 49.1 mg·g-1 at 1.2 V. In the case of MoS2/MXene-1, excess MoS2 nanosheets result in self-aggregation, reducing the MoS2 edges. So, the desalination capacity of MoS2/MXene-4 devices is 44.2 mg·g-1 at 1.2 V. Therefore, MoS2/MXene-2 shows the best desalination performance due to the uniform distribution of MoS2 nanosheets and conductive and hydrophilic MXene substrate.
The Kim-Yoon-Ragone method is employed for further study of the kinetical performance of MXene and hierarchical MoS2/MXene heterostructure devices for CDI systems. As seen in Figure 8, the average desalination rate rises with the voltage escalation at the same initial conductivity, and the average desalination rate also climbs with the initial conductivity at the same voltage. Besides, the desalination rates of the hierarchical MoS2/MXene heterostructure devices are much higher than those of the MXene device. The maximum desalination rate of MoS2/MXene-2 devices reaches 15.2 mg·g-1·min-1 at 1 V, while the desalination rates of MXene, MoS2/MXene-1, and MoS2/MXene-4 devices are 6.1, 12.3, and
CONCLUSIONS
Ti3C2Tx MXene nanosheets were utilized as a conductive and wettable skeleton for fabricating hierarchical MoS2/MXene heterostructures. In the hierarchical MoS2/MXene heterostructures, the pillar-supported MoS2 nanosheets play a critical role in preventing restacking of MXene and enlarging the interlayer spacing of MXene to enhance ion storage capacity. Additionally, the in situ anchoring effect of MXene effectively prevents restacking of MoS2 nanosheets and increases MoS2 active edges. The hierarchical MoS2/MXene heterostructure with well-distributed and active edge-rich MoS2 nanosheets demonstrates a high specific capacitance of 171.8 F·g-1 at 2 A·g-1. Moreover, the optimized MoS2/MXene heterostructure device for CDI exhibits an excellent desalination capacity of
DECLARATIONS
Authors’ contributions
Funding acquisition, resources, methodology, formal analysis, data curation, writing-original draft, review and editing: Zhang Y
Methodology, formal analysis, investigation, validation, data curation: Li X
Formal analysis: Xi W
Data curation: Zhang Q
Data curation: Ge X
Writing-review and editing: You J, Zhang Q, Shi D
Funding acquisition, resources, supervision, writing-review and editing: Jin J
Availability of data and materials
Not applicable.
Financial support and sponsorship
The authors gratefully acknowledge the financial support from the National Natural Science Foundation of China (Nos. 51903040 and 52203340), Scientific Research Program of Department of Education in Hubei Province, and Overseas Expertise Introduction Center for Discipline Innovation (No. D18025).
Conflicts of interest
All authors declared that there are no conflicts of interest.
Ethical approval and consent to participate
Not applicable.
Consent for publication
Not applicable.
Copyright
© The Author(s) 2024.
Supplementary Materials
REFERENCES
1. Deng D, Aouad W, Braff WA, Schlumpberger S, Suss ME, Bazant MZ. Water purification by shock electrodialysis: deionization, filtration, separation, and disinfection. Desalination 2015;357:77-83.
2. Xu X, Allah AE, Wang C, et al. Capacitive deionization using nitrogen-doped mesostructured carbons for highly efficient brackish water desalination. Chem Eng J 2019;362:887-96.
3. Zhao D, Lee LY, Ong SL, Chowdhury P, Siah KB, Ng HY. Electrodialysis reversal for industrial reverse osmosis brine treatment. Sep Purif Technol 2019;213:339-47.
4. Xi W, Jin J, Zhang Y, et al. Hierarchical MXene/transition metal oxide heterostructures for rechargeable batteries, capacitors, and capacitive deionization. Nanoscale 2022;14:11923-44.
5. Cai Y, Zhang L, Fang R, Wang Y, Wang J. Maximized ion accessibility in the binder-free layer-by-layer MXene/CNT film prepared by the electrophoretic deposition for rapid hybrid capacitive deionization. Sep Purif Technol 2022;292:121019.
6. Vafakhah S, Beiramzadeh Z, Saeedikhani M, Yang HY. A review on free-standing electrodes for energy-effective desalination: recent advances and perspectives in capacitive deionization. Desalination 2020;493:114662.
7. Chen B, Feng A, Deng R, Liu K, Yu Y, Song L. MXene as a cation-selective cathode material for asymmetric capacitive deionization. ACS Appl Mater Interfaces 2020;12:13750-8.
8. Zhang W, Liu Y, Jin C, et al. Efficient capacitive deionization with 2D/3D heterostructured carbon electrode derived from chitosan and g-C3N4 nanosheets. Desalination 2022;538:115933.
9. Zhu W, Lin Y, Kang W, et al. An aerogel adsorbent with bio-inspired interfacial adhesion between graphene and MoS2 sheets for water treatment. Appl Surf Sci 2020;512:145717.
10. Xu L, Ding Z, Chen Y, et al. Carbon nanotube bridged nickel hexacyanoferrate architecture for high-performance hybrid capacitive deionization. J Colloid Interface Sci 2023;630:372-81.
11. Tan Z, Wang W, Zhu M, et al. Ti3C2Tx MXene@carbon dots hybrid microflowers as a binder-free electrode material toward high capacity capacitive deionization. Desalination 2023;548:116267.
12. Cai Y, Zhang W, Fang R, Zhao D, Wang Y, Wang J. Well-dispersed few-layered MoS2 connected with robust 3D conductive architecture for rapid capacitive deionization process and its specific ion selectivity. Desalination 2021;520:115325.
13. Cai Y, Zhang W, Zhao J, Wang Y. Flexible structural construction of the ternary composite Ni,Co-Prussian blue analogue@MXene/polypyrrole for high-capacity capacitive deionization. Appl Surf Sci 2023;622:156926.
14. El-deen AG, El-kholly HK, Ali MEM, et al. Polystyrene sulfonate coated activated graphene aerogel for boosting desalination performance using capacitive deionization. Sep Purif Technol 2022;299:121760.
15. Yang X, Jiang H, Zhang W, et al. A novel “butter-sandwich” Ti3C2Tx/PANI/PPY electrode with enhanced adsorption capacity and recyclability toward asymmetric capacitive deionization. Sep Purif Technol 2021;276:119379.
16. Wang H, Wei D, Gang H, et al. Hierarchical porous carbon from the synergistic “pore-on-pore” strategy for efficient capacitive deionization. ACS Sustainable Chem Eng 2020;8:1129-36.
17. Liu Y, Wang K, Xu X, et al. Recent advances in faradic electrochemical deionization: system architectures versus electrode materials. ACS Nano 2021;15:13924-42.
18. Dixit F, Zimmermann K, Dutta R, et al. Application of MXenes for water treatment and energy-efficient desalination: a review. J Hazard Mater 2022;423:127050.
19. Lei J, Xiong Y, Yu F, Ma J. Flexible self-supporting CoFe-LDH/MXene film as a chloride ions storage electrode in capacitive deionization. Chem Eng J 2022;437:135381.
20. Srimuk P, Halim J, Lee J, Tao Q, Rosen J, Presser V. Two-dimensional molybdenum carbide (MXene) with divacancy ordering for brackish and seawater desalination via cation and anion intercalation. ACS Sustainable Chem Eng 2018;6:3739-47.
21. Zhu G, Chen L, Lu T, et al. Cu-based MOF-derived architecture with Cu/Cu2O nanospheres anchored on porous carbon nanosheets for efficient capacitive deionization. Environ Res 2022;210:112909.
22. Yu L, Tao X, Feng S, et al. Recent development of three-dimension printed graphene oxide and MXene-based energy storage devices. Tungsten 2024;6:196-211.
23. Ihsanullah I. MXenes (two-dimensional metal carbides) as emerging nanomaterials for water purification: progress, challenges and prospects. Chem Eng J 2020;388:124340.
24. Li B, Sun K, Xu W, et al. Tailoring interlayer spacing in MXene cathodes to boost the desalination performance of hybrid capacitive deionization systems. Nano Res 2023;16:6039-47.
25. Wang S, Li Z, Wang G, Wang Y, Ling Z, Li C. Freestanding Ti3C2Tx MXene/Prussian blue analogues films with superior ion uptake for efficient capacitive deionization by a dual pseudocapacitance effect. ACS Nano 2022;16:1239-49.
26. Qie H, Liu M, Fu X, et al. Interfacial charge-modulated multifunctional MoS2/Ti3C2Tx penetrating electrode for high-efficiency freshwater production. ACS Nano 2022;16:18898-909.
27. Wang K, Chen L, Zhu G, et al. Ferroferric oxide@titanium carbide MXene heterostructure with enhanced sodium storage ability for efficient hybrid capacitive deionization. Desalination 2022;522:115420.
28. Gong S, Liu H, Zhao F, et al. Vertically aligned bismuthene nanosheets on MXene for high-performance capacitive deionization. ACS Nano 2023;17:4843-53.
29. Mateen A, Ahmad Z, Ali S, et al. Silicon intercalation on MXene nanosheets towards new insights into a superior electrode material for high-performance Zn-ion supercapacitor. J Energy Storage 2023;71:108151.
30. Chen Z, Xu X, Wang K, et al. Hybrid of pyrazine based π-conjugated organic molecule and MXene for hybrid capacitive deionization. Sep Purif Technol 2023;315:123628.
31. Wang L, Liu Z, Wang Z, et al. Up-shifting the desalination rate limit of capacitive deionization via integrating chloride-capturing Bi nanocluster with flow-through cell architecture. Chem Eng J 2023;460:141726.
32. Chen Z, Xu X, Liu Y, et al. Ultra-durable and highly-efficient hybrid capacitive deionization by MXene confined MoS2 heterostructure. Desalination 2022;528:115616.
33. Hao Z, Cai Y, Wang Y, Xu S, Wang J. A coupling technology of capacitive deionization and MoS2/nitrogen-doped carbon spheres with abundant active sites for efficiently and selectively adsorbing low-concentration copper ions. J Colloid Interface Sci 2020;564:428-41.
34. Liu Y, Du X, Wang Z, et al. MoS2 nanoflakes-coated electrospun carbon nanofibers for “rocking-chair” capacitive deionization. Desalination 2021;520:115376.
35. Nguyen TKA, Wang TH, Doong R. Architectures of flower-like MoS2 nanosheet coated N-doped carbon sphere electrode materials for enhanced capacitive deionization. Desalination 2022;540:115979.
36. Peng W, Wang W, Han G, Huang Y, Zhang Y. Fabrication of 3D flower-like MoS2/graphene composite as high-performance electrode for capacitive deionization. Desalination 2020;473:114191.
37. Tian S, Zhang X, Zhang Z. Novel MoS2/NOMC electrodes with enhanced capacitive deionization performances. Chem Eng J 2021;409:128200.
Cite This Article
Export citation file: BibTeX | RIS
OAE Style
Zhang Y, Li X, Xi W, Zhang Q, Ge X, You J, Zhang Q, Shi D, Jin J. Constructing a hierarchical MoS2/MXene heterostructure for efficient capacitive deionization of saline water. Chem Synth 2024;4:19. http://dx.doi.org/10.20517/cs.2023.37
AMA Style
Zhang Y, Li X, Xi W, Zhang Q, Ge X, You J, Zhang Q, Shi D, Jin J. Constructing a hierarchical MoS2/MXene heterostructure for efficient capacitive deionization of saline water. Chemical Synthesis. 2024; 4(2): 19. http://dx.doi.org/10.20517/cs.2023.37
Chicago/Turabian Style
Zhang, Youfang, Xin Li, Wen Xi, Qi Zhang, Xiaohui Ge, Jun You, Qunchao Zhang, Dean Shi, Jun Jin. 2024. "Constructing a hierarchical MoS2/MXene heterostructure for efficient capacitive deionization of saline water" Chemical Synthesis. 4, no.2: 19. http://dx.doi.org/10.20517/cs.2023.37
ACS Style
Zhang, Y.; Li X.; Xi W.; Zhang Q.; Ge X.; You J.; Zhang Q.; Shi D.; Jin J. Constructing a hierarchical MoS2/MXene heterostructure for efficient capacitive deionization of saline water. Chem. Synth. 2024, 4, 19. http://dx.doi.org/10.20517/cs.2023.37
About This Article
Copyright
Author Biographies
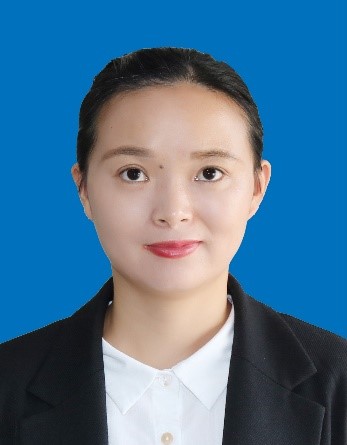
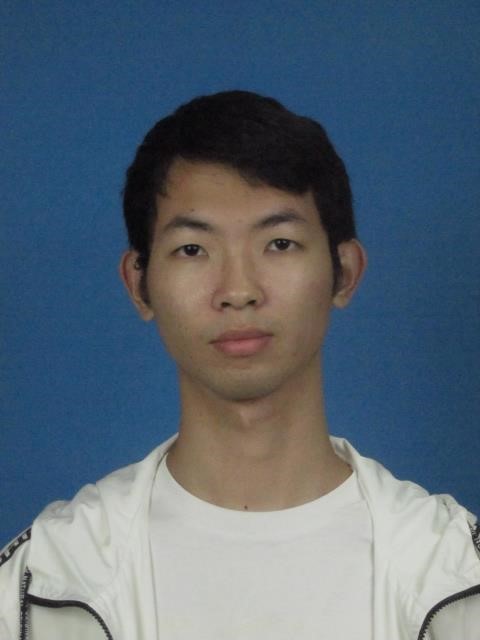
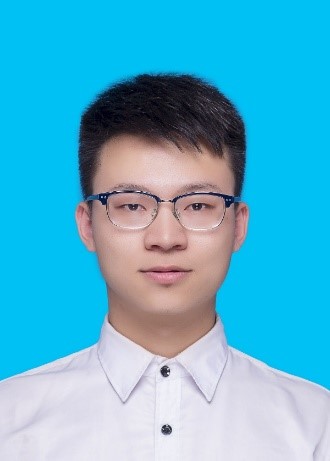
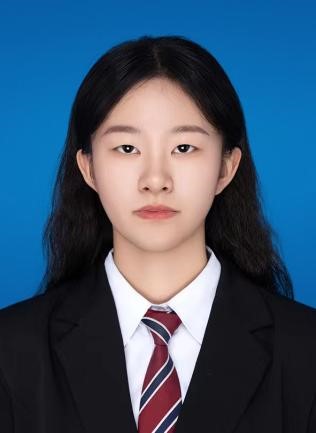
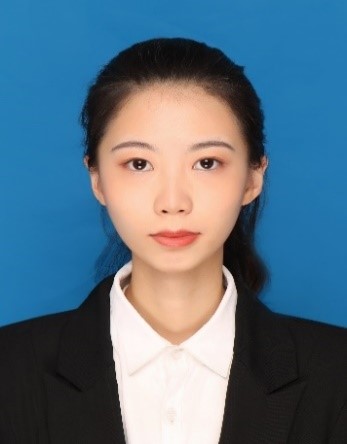
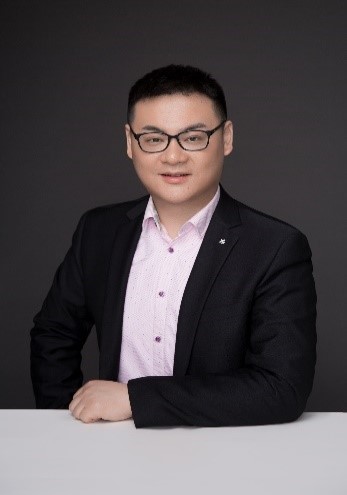
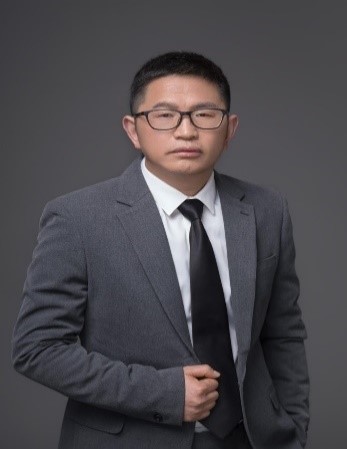
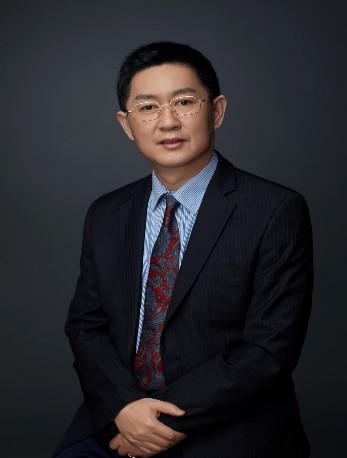
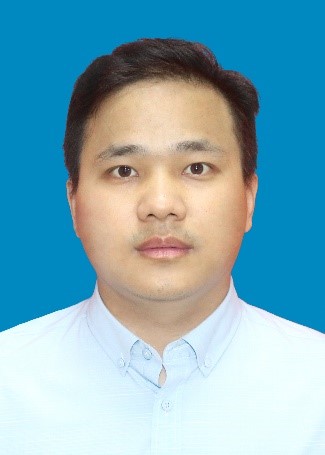
Data & Comments
Data
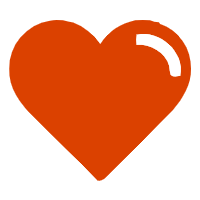

Comments
Comments must be written in English. Spam, offensive content, impersonation, and private information will not be permitted. If any comment is reported and identified as inappropriate content by OAE staff, the comment will be removed without notice. If you have any queries or need any help, please contact us at support@oaepublish.com.